Routine Optical Clearing of 3D-Cell Cultures: Simplicity Forward
- PMID: 32154265
- PMCID: PMC7046628
- DOI: 10.3389/fmolb.2020.00020
Routine Optical Clearing of 3D-Cell Cultures: Simplicity Forward
Abstract
Three-dimensional cell cultures, such as spheroids and organoids, serve as increasingly important models in fundamental and applied research and start to be used for drug screening purposes. Optical tissue clearing procedures are employed to enhance visualization of fluorescence-stained organs, tissues, and three-dimensional cell cultures. To get a more systematic overview about the effects and applicability of optical tissue clearing on three-dimensional cell cultures, we compared six different clearing/embedding protocols on seven types of spheroid- and chip-based three-dimensional cell cultures of approximately 300 μm in size that were stained with nuclear dyes, immunofluorescence, cell trackers, and cyan fluorescent protein. Subsequent whole mount confocal microscopy and semi-automated image analysis were performed to quantify the effects. Quantitative analysis included fluorescence signal intensity and signal-to-noise ratio as a function of z-depth as well as segmentation and counting of nuclei and immunopositive cells. In general, these analyses revealed five key points, which largely confirmed current knowledge and were quantified in this study. First, there was a massive variability of effects of different clearing protocols on sample transparency and shrinkage as well as on dye quenching. Second, all tested clearing protocols worked more efficiently on samples prepared with one cell type than on co-cultures. Third, z-compensation was imperative to minimize variations in signal-to-noise ratio. Fourth, a combination of sample-inherent cell density, sample shrinkage, uniformity of signal-to-noise ratio, and image resolution had a strong impact on data segmentation, cell counts, and relative numbers of immunofluorescence-positive cells. Finally, considering all mentioned aspects and including a wish for simplicity and speed of protocols - in particular, for screening purposes - clearing with 88% Glycerol appeared to be the most promising option amongst the ones tested.
Keywords: glycerol; optical tissue clearing; organoid; spheroid; z-compensation.
Copyright © 2020 Nürnberg, Vitacolonna, Klicks, von Molitor, Cesetti, Keller, Bruch, Ertongur-Fauth, Riedel, Scholz, Lau, Schneider, Meier, Hafner and Rudolf.
Figures
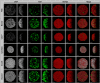
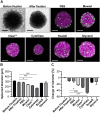
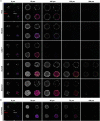
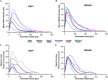
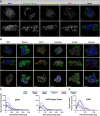
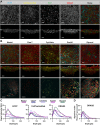
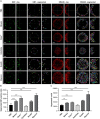
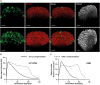
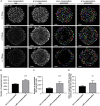
Similar articles
-
Quantitative Analysis of Whole-Mount Fluorescence-Stained Tumor Spheroids in Phenotypic Drug Screens.Methods Mol Biol. 2024;2764:311-334. doi: 10.1007/978-1-0716-3674-9_20. Methods Mol Biol. 2024. PMID: 38393603
-
HyClear: A Novel Tissue Clearing Solution for One-Step Clearing of Microtissues.Cells. 2022 Nov 30;11(23):3854. doi: 10.3390/cells11233854. Cells. 2022. PMID: 36497111 Free PMC article.
-
On-chip clearing of arrays of 3-D cell cultures and micro-tissues.Biomicrofluidics. 2016 Jul 20;10(4):044107. doi: 10.1063/1.4959031. eCollection 2016 Jul. Biomicrofluidics. 2016. PMID: 27493703 Free PMC article.
-
Current Status of Tissue Clearing and the Path Forward in Neuroscience.ACS Chem Neurosci. 2021 Jan 6;12(1):5-29. doi: 10.1021/acschemneuro.0c00563. Epub 2020 Dec 16. ACS Chem Neurosci. 2021. PMID: 33326739 Review.
-
Can Developments in Tissue Optical Clearing Aid Super-Resolution Microscopy Imaging?Int J Mol Sci. 2021 Jun 23;22(13):6730. doi: 10.3390/ijms22136730. Int J Mol Sci. 2021. PMID: 34201632 Free PMC article. Review.
Cited by
-
Assay establishment and validation of a high-throughput organoid-based drug screening platform.Stem Cell Res Ther. 2022 May 26;13(1):219. doi: 10.1186/s13287-022-02902-3. Stem Cell Res Ther. 2022. PMID: 35619149 Free PMC article.
-
Synthesis of large scale 3D microscopic images of 3D cell cultures for training and benchmarking.PLoS One. 2023 Mar 31;18(3):e0283828. doi: 10.1371/journal.pone.0283828. eCollection 2023. PLoS One. 2023. PMID: 37000778 Free PMC article.
-
Aggregation of human osteoblasts unlocks self-reliant differentiation and constitutes a microenvironment for 3D-co-cultivation with other bone marrow cells.Sci Rep. 2024 May 6;14(1):10345. doi: 10.1038/s41598-024-60986-8. Sci Rep. 2024. PMID: 38710795 Free PMC article.
-
Factors to consider when interrogating 3D culture models with plate readers or automated microscopes.In Vitro Cell Dev Biol Anim. 2021 Feb;57(2):238-256. doi: 10.1007/s11626-020-00537-3. Epub 2021 Feb 9. In Vitro Cell Dev Biol Anim. 2021. PMID: 33564998 Free PMC article. Review.
-
Optimization of a Three-Dimensional Culturing Method for Assessing the Impact of Cisplatin on Notch Signaling in Head and Neck Squamous Cell Carcinoma (HNSCC).Cancers (Basel). 2023 Nov 7;15(22):5320. doi: 10.3390/cancers15225320. Cancers (Basel). 2023. PMID: 38001580 Free PMC article.
References
LinkOut - more resources
Full Text Sources
Other Literature Sources