Mitochondrial function in skeletal myofibers is controlled by a TRF2-SIRT3 axis over lifetime
- PMID: 31991048
- PMCID: PMC7059141
- DOI: 10.1111/acel.13097
Mitochondrial function in skeletal myofibers is controlled by a TRF2-SIRT3 axis over lifetime
Abstract
Telomere shortening follows a developmentally regulated process that leads to replicative senescence of dividing cells. However, whether telomere changes are involved in postmitotic cell function and aging remains elusive. In this study, we discovered that the level of the TRF2 protein, a key telomere-capping protein, declines in human skeletal muscle over lifetime. In cultured human myotubes, TRF2 downregulation did not trigger telomere dysfunction, but suppressed expression of the mitochondrial Sirtuin 3 gene (SIRT3) leading to mitochondrial respiration dysfunction and increased levels of reactive oxygen species. Importantly, restoring the Sirt3 level in TRF2-compromised myotubes fully rescued mitochondrial functions. Finally, targeted ablation of the Terf2 gene in mouse skeletal muscle leads to mitochondrial dysfunction and sirt3 downregulation similarly to those of TRF2-compromised human myotubes. Altogether, these results reveal a TRF2-SIRT3 axis controlling muscle mitochondrial function. We propose that this axis connects developmentally regulated telomere changes to muscle redox metabolism.
Keywords: aging; mitochondria; postmitotic cells; skeletal muscle; telomeres.
© 2020 The Authors. Aging Cell published by the Anatomical Society and John Wiley & Sons Ltd.
Conflict of interest statement
None declared.
Figures
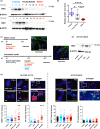
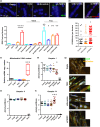
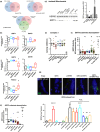
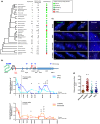
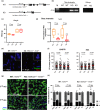
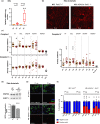
Similar articles
-
Non-canonical telomere protection role of FOXO3a of human skeletal muscle cells regulated by the TRF2-redox axis.Commun Biol. 2023 May 25;6(1):561. doi: 10.1038/s42003-023-04903-1. Commun Biol. 2023. PMID: 37231173 Free PMC article.
-
Telomeres shorten in response to oxidative stress in mouse skeletal muscle fibers.J Gerontol A Biol Sci Med Sci. 2014 Jul;69(7):821-30. doi: 10.1093/gerona/glt211. Epub 2014 Jan 13. J Gerontol A Biol Sci Med Sci. 2014. PMID: 24418792 Free PMC article.
-
Role of the telomeric factor TRF2 in post-hypoxic brain damages.Redox Biol. 2024 Sep;75:103278. doi: 10.1016/j.redox.2024.103278. Epub 2024 Jul 25. Redox Biol. 2024. PMID: 39128227 Free PMC article.
-
Linking telomere loss and mitochondrial dysfunction in chronic disease.Front Biosci (Landmark Ed). 2017 Jan 1;22(1):117-127. doi: 10.2741/4475. Front Biosci (Landmark Ed). 2017. PMID: 27814605 Review.
-
Interstitial telomeric loops and implications of the interaction between TRF2 and lamin A/C.Differentiation. 2018 Jul-Aug;102:19-26. doi: 10.1016/j.diff.2018.06.001. Epub 2018 Jun 18. Differentiation. 2018. PMID: 29979997 Review.
Cited by
-
RNA-Seq-based discovery of genetic variants and allele-specific expression of two layer lines and broiler chicken.Evol Appl. 2023 May 22;16(6):1135-1153. doi: 10.1111/eva.13557. eCollection 2023 Jun. Evol Appl. 2023. PMID: 37360029 Free PMC article.
-
The non-telomeric evolutionary trajectory of TRF2 in zebrafish reveals its specific roles in neurodevelopment and aging.Nucleic Acids Res. 2022 Feb 28;50(4):2081-2095. doi: 10.1093/nar/gkac065. Nucleic Acids Res. 2022. PMID: 35150283 Free PMC article.
-
Biomarkers of aging.Sci China Life Sci. 2023 May;66(5):893-1066. doi: 10.1007/s11427-023-2305-0. Epub 2023 Apr 11. Sci China Life Sci. 2023. PMID: 37076725 Free PMC article. Review.
-
TIN2 modulates FOXO1 mitochondrial shuttling to enhance oxidative stress-induced apoptosis in retinal pigment epithelium under hyperglycemia.Cell Death Differ. 2024 Nov;31(11):1487-1505. doi: 10.1038/s41418-024-01349-8. Epub 2024 Jul 30. Cell Death Differ. 2024. PMID: 39080375
-
Calorie restriction modulates the transcription of genes related to stress response and longevity in human muscle: The CALERIE study.Aging Cell. 2023 Dec;22(12):e13963. doi: 10.1111/acel.13963. Epub 2023 Oct 12. Aging Cell. 2023. PMID: 37823711 Free PMC article. Clinical Trial.
References
-
- Ahmed, S. , Passos, J. F. , Birket, M. J. , Beckmann, T. , Brings, S. , Peters, H. , … Saretzki, G. (2008). Telomerase does not counteract telomere shortening but protects mitochondrial function under oxidative stress. Journal of Cell Science, 121, 1046–1053. - PubMed
Publication types
MeSH terms
Substances
LinkOut - more resources
Full Text Sources
Medical
Molecular Biology Databases
Research Materials
Miscellaneous