Janus particles: recent advances in the biomedical applications
- PMID: 31692550
- PMCID: PMC6711559
- DOI: 10.2147/IJN.S169030
Janus particles: recent advances in the biomedical applications
Abstract
Janus particles, which are named after the two-faced Roman god Janus, have two distinct sides with different surface features, structures, and compositions. This asymmetric structure enables the combination of different or even incompatible physical, chemical, and mechanical properties within a single particle. Much effort has been focused on the preparation of Janus particles with high homogeneity, tunable size and shape, combined functionalities, and scalability. With their unique features, Janus particles have attracted attention in a wide range of applications such as in optics, catalysis, and biomedicine. As a biomedical device, Janus particles offer opportunities to incorporate therapeutics, imaging, or sensing modalities in independent compartments of a single particle in a spatially controlled manner. This may result in synergistic actions of combined therapies and multi-level targeting not possible in isotropic systems. In this review, we summarize the latest advances in employing Janus particles as therapeutic delivery carriers, in vivo imaging probes, and biosensors. Challenges and future opportunities for these particles will also be discussed.
Keywords: Janus particles; imaging; sensing; theranostics; therapeutics.
© 2019 Le et al.
Conflict of interest statement
The authors report no conflicts of interest in this work.
Figures
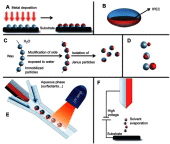
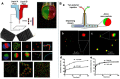
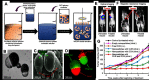
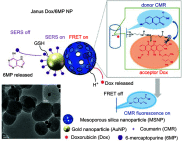
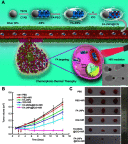
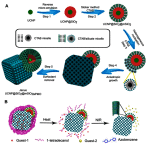
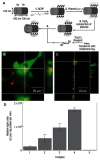
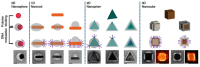
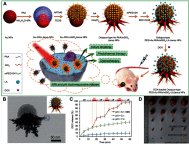
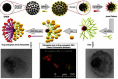
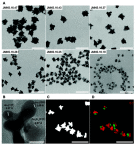
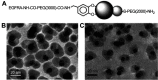
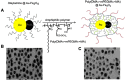
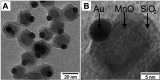
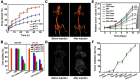
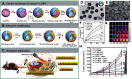
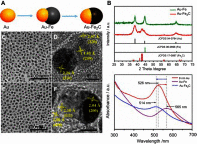
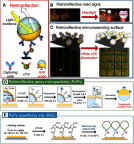
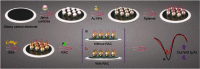
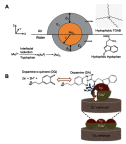
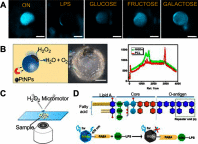
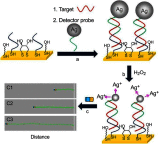
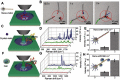
Similar articles
-
Application of Janus Particles in Point-of-Care Testing.Biosensors (Basel). 2022 Aug 26;12(9):689. doi: 10.3390/bios12090689. Biosensors (Basel). 2022. PMID: 36140074 Free PMC article. Review.
-
Janus particles for biological imaging and sensing.Analyst. 2016 Jun 21;141(12):3526-39. doi: 10.1039/c6an00325g. Epub 2016 Apr 7. Analyst. 2016. PMID: 27052001 Free PMC article. Review.
-
Magnetic nanoparticles: A multifunctional vehicle for modern theranostics.Biochim Biophys Acta Gen Subj. 2017 Jun;1861(6):1642-1651. doi: 10.1016/j.bbagen.2017.02.022. Epub 2017 Feb 20. Biochim Biophys Acta Gen Subj. 2017. PMID: 28219721 Review.
-
Bioengineering of Metal-organic Frameworks for Nanomedicine.Theranostics. 2019 May 18;9(11):3122-3133. doi: 10.7150/thno.31918. eCollection 2019. Theranostics. 2019. PMID: 31244945 Free PMC article. Review.
-
Anisotropic noble metal nanoparticles: Synthesis, surface functionalization and applications in biosensing, bioimaging, drug delivery and theranostics.Acta Biomater. 2017 Feb;49:45-65. doi: 10.1016/j.actbio.2016.11.066. Epub 2016 Nov 30. Acta Biomater. 2017. PMID: 27915023 Review.
Cited by
-
Stimulus-Responsive Sequential Release Systems for Drug and Gene Delivery.Nano Today. 2020 Oct;34:100914. doi: 10.1016/j.nantod.2020.100914. Epub 2020 Jul 2. Nano Today. 2020. PMID: 32788923 Free PMC article.
-
Application of Janus Particles in Point-of-Care Testing.Biosensors (Basel). 2022 Aug 26;12(9):689. doi: 10.3390/bios12090689. Biosensors (Basel). 2022. PMID: 36140074 Free PMC article. Review.
-
Modular Drug-Loaded Nanocapsules with Metal Dome Layers as a Platform for Obtaining Synergistic Therapeutic Biological Activities.ACS Appl Mater Interfaces. 2023 Nov 1;15(43):50330-50343. doi: 10.1021/acsami.3c07188. Epub 2023 Oct 20. ACS Appl Mater Interfaces. 2023. PMID: 37861446 Free PMC article.
-
A 3D-Printed Standardized Modular Microfluidic System for Droplet Generation.Biosensors (Basel). 2022 Nov 28;12(12):1085. doi: 10.3390/bios12121085. Biosensors (Basel). 2022. PMID: 36551052 Free PMC article.
-
Craft of Co-encapsulation in Nanomedicine: A Struggle To Achieve Synergy through Reciprocity.ACS Pharmacol Transl Sci. 2022 May 2;5(5):278-298. doi: 10.1021/acsptsci.2c00033. eCollection 2022 May 13. ACS Pharmacol Transl Sci. 2022. PMID: 35592431 Free PMC article. Review.
References
Publication types
MeSH terms
Substances
LinkOut - more resources
Full Text Sources
Medical
Research Materials