Near-equilibrium glycolysis supports metabolic homeostasis and energy yield
- PMID: 31548693
- PMCID: PMC10184052
- DOI: 10.1038/s41589-019-0364-9
Near-equilibrium glycolysis supports metabolic homeostasis and energy yield
Abstract
Glycolysis plays a central role in producing ATP and biomass. Its control principles, however, remain incompletely understood. Here, we develop a method that combines 2H and 13C tracers to determine glycolytic thermodynamics. Using this method, we show that, in conditions and organisms with relatively slow fluxes, multiple steps in glycolysis are near to equilibrium, reflecting spare enzyme capacity. In Escherichia coli, nitrogen or phosphorus upshift rapidly increases the thermodynamic driving force, deploying the spare enzyme capacity to increase flux. Similarly, respiration inhibition in mammalian cells rapidly increases both glycolytic flux and the thermodynamic driving force. The thermodynamic shift allows flux to increase with only small metabolite concentration changes. Finally, we find that the cellulose-degrading anaerobe Clostridium cellulolyticum exhibits slow, near-equilibrium glycolysis due to the use of pyrophosphate rather than ATP for fructose-bisphosphate production, resulting in enhanced per-glucose ATP yield. Thus, near-equilibrium steps of glycolysis promote both rapid flux adaptation and energy efficiency.
Conflict of interest statement
Competing interests
The authors declare no competing interests.
Figures
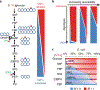
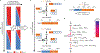
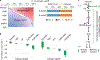
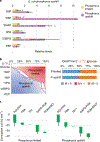
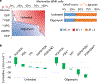
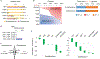
Similar articles
-
Increasing the Thermodynamic Driving Force of the Phosphofructokinase Reaction in Clostridium thermocellum.Appl Environ Microbiol. 2022 Nov 22;88(22):e0125822. doi: 10.1128/aem.01258-22. Epub 2022 Oct 26. Appl Environ Microbiol. 2022. PMID: 36286488 Free PMC article.
-
In Vivo Thermodynamic Analysis of Glycolysis in Clostridium thermocellum and Thermoanaerobacterium saccharolyticum Using 13C and 2H Tracers.mSystems. 2020 Mar 17;5(2):e00736-19. doi: 10.1128/mSystems.00736-19. mSystems. 2020. PMID: 32184362 Free PMC article.
-
Regulation of glycolytic flux and overflow metabolism depending on the source of energy generation for energy demand.Biotechnol Adv. 2019 Mar-Apr;37(2):284-305. doi: 10.1016/j.biotechadv.2018.12.007. Epub 2018 Dec 18. Biotechnol Adv. 2019. PMID: 30576718 Review.
-
The effect of substrate cycling on the ATP yield of sperm glycolysis.J Biol Chem. 1983 Jul 25;258(14):8759-68. J Biol Chem. 1983. PMID: 6863309
-
Mathematical models for explaining the Warburg effect: a review focussed on ATP and biomass production.Biochem Soc Trans. 2015 Dec;43(6):1187-94. doi: 10.1042/BST20150153. Biochem Soc Trans. 2015. PMID: 26614659 Review.
Cited by
-
MR1-Restricted T Cells in Cancer Immunotherapy.Cancers (Basel). 2020 Aug 3;12(8):2145. doi: 10.3390/cancers12082145. Cancers (Basel). 2020. PMID: 32756356 Free PMC article. Review.
-
Extracellular Metabolism Sets the Table for Microbial Cross-Feeding.Microbiol Mol Biol Rev. 2021 Jan 13;85(1):e00135-20. doi: 10.1128/MMBR.00135-20. Print 2021 Feb 17. Microbiol Mol Biol Rev. 2021. PMID: 33441489 Free PMC article. Review.
-
Increasing the Thermodynamic Driving Force of the Phosphofructokinase Reaction in Clostridium thermocellum.Appl Environ Microbiol. 2022 Nov 22;88(22):e0125822. doi: 10.1128/aem.01258-22. Epub 2022 Oct 26. Appl Environ Microbiol. 2022. PMID: 36286488 Free PMC article.
-
Metabolic regulation of the hallmarks of stem cell biology.Cell Stem Cell. 2024 Feb 1;31(2):161-180. doi: 10.1016/j.stem.2024.01.003. Cell Stem Cell. 2024. PMID: 38306993 Free PMC article. Review.
-
Modular dynamic biomolecular modelling with bond graphs: the unification of stoichiometry, thermodynamics, kinetics and data.J R Soc Interface. 2021 Aug;18(181):20210478. doi: 10.1098/rsif.2021.0478. Epub 2021 Aug 25. J R Soc Interface. 2021. PMID: 34428949 Free PMC article.
References
-
- Fell D Understanding the Control of Metabolism (Portland Press, 1997).