Dynamic postnatal development of the cellular and circuit properties of striatal D1 and D2 spiny projection neurons
- PMID: 31531863
- PMCID: PMC6900874
- DOI: 10.1113/JP278416
Dynamic postnatal development of the cellular and circuit properties of striatal D1 and D2 spiny projection neurons
Abstract
Key points: Imbalances in the activity of the D1-expressing direct pathway and D2-expressing indirect pathway striatal projection neurons (SPNs) are thought to contribute to many basal ganglia disorders, including early-onset neurodevelopmental disorders such as obsessive-compulsive disorder, attention deficit hyperactivity disorder and Tourette's syndrome. This study provides the first detailed quantitative investigation of development of D1 and D2 SPNs, including their cellular properties and connectivity within neural circuits, during the first postnatal weeks. This period is highly dynamic with many properties changing, but it is possible to make three main observations: many aspects of D1 and D2 SPNs progressively mature in parallel; there are notable exceptions when they diverge; and many of the defining properties of mature striatal SPNs and circuits are already established by the first and second postnatal weeks, suggesting guidance through intrinsic developmental programmes. These findings provide an experimental framework for future studies of striatal development in both health and disease.
Abstract: Many basal ganglia neurodevelopmental disorders are thought to result from imbalances in the activity of the D1-expressing direct pathway and D2-expressing indirect pathway striatal projection neurons (SPNs). Insight into these disorders is reliant on our understanding of normal D1 and D2 SPN development. Here we provide the first detailed study and quantification of the striatal cellular and circuit changes occurring for both D1 and D2 SPNs in the first postnatal weeks using in vitro whole-cell patch-clamp electrophysiology. Characterization of their intrinsic electrophysiological and morphological properties, the excitatory long-range inputs coming from cortex and thalamus, as well their local gap junction and inhibitory synaptic connections reveals this period to be highly dynamic with numerous properties changing. However it is possible to make three main observations. Firstly, many aspects of SPNs mature in parallel, including intrinsic membrane properties, increases in dendritic arbours and spine densities, general synaptic inputs and expression of specific glutamate receptors. Secondly, there are notable exceptions, including a transient stronger thalamic innervation of D2 SPNs and stronger cortical NMDA receptor-mediated inputs to D1 SPNs, both in the second postnatal week. Thirdly, many of the defining properties of mature D1 and D2 SPNs and striatal circuits are already established by the first and second postnatal weeks, including different electrophysiological properties as well as biased local inhibitory connections between SPNs, suggesting this is guided through intrinsic developmental programmes. Together these findings provide an experimental framework for future studies of D1 and D2 SPN development in health and disease.
© 2019 The Authors. The Journal of Physiology published by John Wiley & Sons Ltd on behalf of The Physiological Society.
Figures
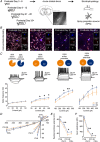
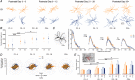
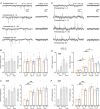
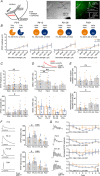
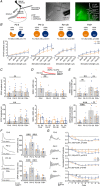
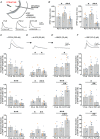
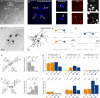
Similar articles
-
Altered Development of Synapse Structure and Function in Striatum Caused by Parkinson's Disease-Linked LRRK2-G2019S Mutation.J Neurosci. 2016 Jul 6;36(27):7128-41. doi: 10.1523/JNEUROSCI.3314-15.2016. J Neurosci. 2016. PMID: 27383589 Free PMC article.
-
Selective Vulnerability of Striatal D2 versus D1 Dopamine Receptor-Expressing Medium Spiny Neurons in HIV-1 Tat Transgenic Male Mice.J Neurosci. 2017 Jun 7;37(23):5758-5769. doi: 10.1523/JNEUROSCI.0622-17.2017. Epub 2017 May 4. J Neurosci. 2017. PMID: 28473642 Free PMC article.
-
Differential electrophysiological properties of D1 and D2 spiny projection neurons in the mouse nucleus accumbens core.Physiol Rep. 2018 Jul;6(13):e13784. doi: 10.14814/phy2.13784. Physiol Rep. 2018. PMID: 29962016 Free PMC article.
-
D1 and D2 dopamine-receptor modulation of striatal glutamatergic signaling in striatal medium spiny neurons.Trends Neurosci. 2007 May;30(5):228-35. doi: 10.1016/j.tins.2007.03.008. Epub 2007 Apr 3. Trends Neurosci. 2007. PMID: 17408758 Review.
-
Striatal D2 receptors and LTD: yes, but not where you thought they were.Neuron. 2006 May 4;50(3):347-8. doi: 10.1016/j.neuron.2006.04.023. Neuron. 2006. PMID: 16675388 Review.
Cited by
-
Developmental regulation of thalamus-driven pauses in striatal cholinergic interneurons.iScience. 2022 Oct 13;25(11):105332. doi: 10.1016/j.isci.2022.105332. eCollection 2022 Nov 18. iScience. 2022. PMID: 36325074 Free PMC article.
-
Post-transcriptional regulation and subcellular localization of G-protein γ7 subunit: implications for striatal function and behavioral responses to cocaine.Front Neuroanat. 2024 May 2;18:1394659. doi: 10.3389/fnana.2024.1394659. eCollection 2024. Front Neuroanat. 2024. PMID: 38764487 Free PMC article.
-
Generation and propagation of bursts of activity in the developing basal ganglia.Cereb Cortex. 2023 Oct 9;33(20):10595-10613. doi: 10.1093/cercor/bhad307. Cereb Cortex. 2023. PMID: 37615347 Free PMC article.
-
Dynamic proteomic and phosphoproteomic atlas of corticostriatal axons in neurodevelopment.Elife. 2022 Oct 14;11:e78847. doi: 10.7554/eLife.78847. Elife. 2022. PMID: 36239373 Free PMC article.
-
Histamine, Neuroinflammation and Neurodevelopment: A Review.Front Neurosci. 2021 Jul 14;15:680214. doi: 10.3389/fnins.2021.680214. eCollection 2021. Front Neurosci. 2021. PMID: 34335160 Free PMC article. Review.
References
-
- Akerman CJ, Smyth D & Thompson ID (2002). Visual experience before eye‐opening and the development of the retinogeniculate pathway. Neuron 36, 869–879. - PubMed
-
- Bagetta V, Picconi B, Marinucci S, Sgobio C, Pendolino V, Ghiglieri V, Fusco FR, Giampa C & Calabresi P (2011). Dopamine‐dependent long‐term depression is expressed in striatal spiny neurons of both direct and indirect pathways: implications for Parkinson's disease. J Neurosci 31, 12513–12522. - PMC - PubMed
Publication types
MeSH terms
Substances
Grants and funding
LinkOut - more resources
Full Text Sources
Molecular Biology Databases
Miscellaneous