The long non-coding RNA Cerox1 is a post transcriptional regulator of mitochondrial complex I catalytic activity
- PMID: 31045494
- PMCID: PMC6542586
- DOI: 10.7554/eLife.45051
The long non-coding RNA Cerox1 is a post transcriptional regulator of mitochondrial complex I catalytic activity
Erratum in
-
Correction: The long non-coding RNA Cerox1 is a post transcriptional regulator of mitochondrial complex I catalytic activity.Elife. 2019 Aug 12;8:e50980. doi: 10.7554/eLife.50980. Elife. 2019. PMID: 31403403 Free PMC article.
Abstract
To generate energy efficiently, the cell is uniquely challenged to co-ordinate the abundance of electron transport chain protein subunits expressed from both nuclear and mitochondrial genomes. How an effective stoichiometry of this many constituent subunits is co-ordinated post-transcriptionally remains poorly understood. Here we show that Cerox1, an unusually abundant cytoplasmic long noncoding RNA (lncRNA), modulates the levels of mitochondrial complex I subunit transcripts in a manner that requires binding to microRNA-488-3p. Increased abundance of Cerox1 cooperatively elevates complex I subunit protein abundance and enzymatic activity, decreases reactive oxygen species production, and protects against the complex I inhibitor rotenone. Cerox1 function is conserved across placental mammals: human and mouse orthologues effectively modulate complex I enzymatic activity in mouse and human cells, respectively. Cerox1 is the first lncRNA demonstrated, to our knowledge, to regulate mitochondrial oxidative phosphorylation and, with miR-488-3p, represent novel targets for the modulation of complex I activity.
Keywords: biochemistry; chemical biology; energy metabolism; genetics; genomics; human; long noncoding RNA; miRNA; mitochondria; mouse; post-transcriptional regulation.
© 2019, Sirey et al.
Conflict of interest statement
TS, KR, WH, OB, SR, JT, NL, LH, RC, SC, AF, JW, NM, AM No competing interests declared, CP Reviewing editor, eLife
Figures
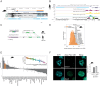
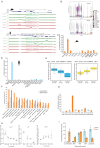
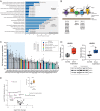
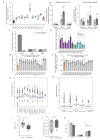
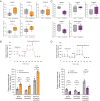
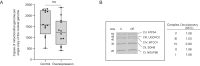
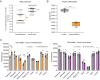
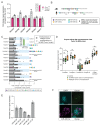
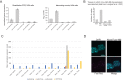
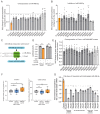
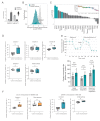
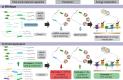
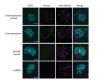
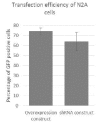
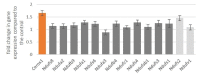
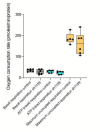
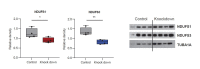
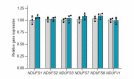
Similar articles
-
Correction: The long non-coding RNA Cerox1 is a post transcriptional regulator of mitochondrial complex I catalytic activity.Elife. 2019 Aug 12;8:e50980. doi: 10.7554/eLife.50980. Elife. 2019. PMID: 31403403 Free PMC article.
-
LncRNA SNHG15 Knockdown Protects Against OGD/R-Induced Neuron Injury by Downregulating TP53INP1 Expression via Binding to miR-455-3p.Neurochem Res. 2021 Apr;46(4):1019-1030. doi: 10.1007/s11064-020-03222-9. Epub 2021 Feb 2. Neurochem Res. 2021. PMID: 33528807
-
Long Noncoding RNA NRAV Promotes Respiratory Syncytial Virus Replication by Targeting the MicroRNA miR-509-3p/Rab5c Axis To Regulate Vesicle Transportation.J Virol. 2020 May 4;94(10):e00113-20. doi: 10.1128/JVI.00113-20. Print 2020 May 4. J Virol. 2020. PMID: 32102886 Free PMC article.
-
Transcriptional and Post-transcriptional Gene Regulation by Long Non-coding RNA.Genomics Proteomics Bioinformatics. 2017 Jun;15(3):177-186. doi: 10.1016/j.gpb.2016.12.005. Epub 2017 May 19. Genomics Proteomics Bioinformatics. 2017. PMID: 28529100 Free PMC article. Review.
-
Insights into the post-transcriptional regulation of the mitochondrial electron transport chain.Biochem Soc Trans. 2016 Oct 15;44(5):1491-1498. doi: 10.1042/BST20160100. Biochem Soc Trans. 2016. PMID: 27911731 Free PMC article. Review.
Cited by
-
A novel lncRNA DFRV plays a dual function in influenza A virus infection.Front Microbiol. 2023 May 25;14:1171423. doi: 10.3389/fmicb.2023.1171423. eCollection 2023. Front Microbiol. 2023. PMID: 37303776 Free PMC article.
-
Long non-coding RNAs in ferroptosis, pyroptosis and necroptosis: from functions to clinical implications in cancer therapy.Front Oncol. 2024 Aug 29;14:1437698. doi: 10.3389/fonc.2024.1437698. eCollection 2024. Front Oncol. 2024. PMID: 39267831 Free PMC article. Review.
-
GATA6-AS1 Regulates Intestinal Epithelial Mitochondrial Functions, and its Reduced Expression is Linked to Intestinal Inflammation and Less Favourable Disease Course in Ulcerative Colitis.J Crohns Colitis. 2023 Jun 16;17(6):960-971. doi: 10.1093/ecco-jcc/jjad006. J Crohns Colitis. 2023. PMID: 36655602 Free PMC article.
-
Analysis of lncRNA in the skeletal muscle of rabbits at different developmental stages.Front Vet Sci. 2022 Sep 21;9:948929. doi: 10.3389/fvets.2022.948929. eCollection 2022. Front Vet Sci. 2022. PMID: 36213392 Free PMC article.
-
Antioxidant Systems, lncRNAs, and Tunneling Nanotubes in Cell Death Rescue from Cigarette Smoke Exposure.Cells. 2022 Jul 23;11(15):2277. doi: 10.3390/cells11152277. Cells. 2022. PMID: 35892574 Free PMC article. Review.
References
-
- Ala U, Karreth FA, Bosia C, Pagnani A, Taulli R, Léopold V, Tay Y, Provero P, Zecchina R, Pandolfi PP. Integrated transcriptional and competitive endogenous RNA networks are cross-regulated in permissive molecular environments. PNAS. 2013;110:7154–7159. doi: 10.1073/pnas.1222509110. - DOI - PMC - PubMed
-
- Alvarez-Fischer D, Fuchs J, Castagner F, Stettler O, Massiani-Beaudoin O, Moya KL, Bouillot C, Oertel WH, Lombès A, Faigle W, Joshi RL, Hartmann A, Prochiantz A. Engrailed protects mouse midbrain dopaminergic neurons against mitochondrial complex I insults. Nature Neuroscience. 2011;14:1260–1266. doi: 10.1038/nn.2916. - DOI - PubMed
Publication types
MeSH terms
Substances
Grants and funding
LinkOut - more resources
Full Text Sources
Molecular Biology Databases
Miscellaneous