Cut-and-Run: A Distinct Mechanism by which V(D)J Recombination Causes Genome Instability
- PMID: 30905508
- PMCID: PMC6509286
- DOI: 10.1016/j.molcel.2019.02.025
Cut-and-Run: A Distinct Mechanism by which V(D)J Recombination Causes Genome Instability
Abstract
V(D)J recombination is essential to generate antigen receptor diversity but is also a potent cause of genome instability. Many chromosome alterations that result from aberrant V(D)J recombination involve breaks at single recombination signal sequences (RSSs). A long-standing question, however, is how such breaks occur. Here, we show that the genomic DNA that is excised during recombination, the excised signal circle (ESC), forms a complex with the recombinase proteins to efficiently catalyze breaks at single RSSs both in vitro and in vivo. Following cutting, the RSS is released while the ESC-recombinase complex remains intact to potentially trigger breaks at further RSSs. Consistent with this, chromosome breaks at RSSs increase markedly in the presence of the ESC. Notably, these breaks co-localize with those found in acute lymphoblastic leukemia patients and occur at key cancer driver genes. We have named this reaction "cut-and-run" and suggest that it could be a significant cause of lymphocyte genome instability.
Keywords: RAG proteins; V(D)J recombination; acute lymphoblastic leukemia; chromosome translocations; double strand breaks; genome instability.
Copyright © 2019 The Author(s). Published by Elsevier Inc. All rights reserved.
Figures
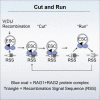
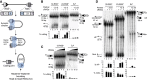
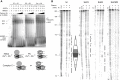
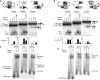
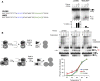
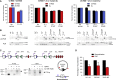
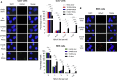
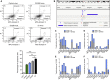
Similar articles
-
The ESC: The Dangerous By-Product of V(D)J Recombination.Front Immunol. 2019 Jul 4;10:1572. doi: 10.3389/fimmu.2019.01572. eCollection 2019. Front Immunol. 2019. PMID: 31333681 Free PMC article. Review.
-
Genome instability triggered by the V(D)J recombination by-product.Mol Cell Oncol. 2019 May 7;6(4):1610323. doi: 10.1080/23723556.2019.1610323. eCollection 2019. Mol Cell Oncol. 2019. PMID: 31211241 Free PMC article.
-
Germline DNA Retention in Murine and Human Rearranged T Cell Receptor Gene Coding Joints: Alternative Recombination Signal Sequences and V(D)J Recombinase Errors.Front Immunol. 2019 Nov 8;10:2637. doi: 10.3389/fimmu.2019.02637. eCollection 2019. Front Immunol. 2019. PMID: 31781122 Free PMC article.
-
V(D)J targeting mistakes occur at low frequency in acute lymphoblastic leukemia.Genes Chromosomes Cancer. 2009 Aug;48(8):725-36. doi: 10.1002/gcc.20677. Genes Chromosomes Cancer. 2009. PMID: 19455608
-
Structural gymnastics of RAG-mediated DNA cleavage in V(D)J recombination.Curr Opin Struct Biol. 2018 Dec;53:178-186. doi: 10.1016/j.sbi.2018.11.001. Epub 2018 Nov 23. Curr Opin Struct Biol. 2018. PMID: 30476719 Free PMC article. Review.
Cited by
-
An instructive role for Interleukin-7 receptor α in the development of human B-cell precursor leukemia.Nat Commun. 2022 Feb 3;13(1):659. doi: 10.1038/s41467-022-28218-7. Nat Commun. 2022. PMID: 35115489 Free PMC article.
-
Evolutionary Comparative Analyses of DNA-Editing Enzymes of the Immune System: From 5-Dimensional Description of Protein Structures to Immunological Insights and Applications to Protein Engineering.Front Immunol. 2021 May 31;12:642343. doi: 10.3389/fimmu.2021.642343. eCollection 2021. Front Immunol. 2021. PMID: 34135887 Free PMC article.
-
DNA crosslinking and recombination-activating genes 1/2 (RAG1/2) are required for oncogenic splicing in acute lymphoblastic leukemia.Cancer Commun (Lond). 2021 Nov;41(11):1116-1136. doi: 10.1002/cac2.12234. Epub 2021 Oct 26. Cancer Commun (Lond). 2021. PMID: 34699692 Free PMC article.
-
Antibody Repertoire Analysis of Tumor-Infiltrating B Cells Reveals Distinct Signatures and Distributions Across Tissues.Front Immunol. 2021 Jul 19;12:705381. doi: 10.3389/fimmu.2021.705381. eCollection 2021. Front Immunol. 2021. PMID: 34349765 Free PMC article.
-
The multifaceted functions of DNA-PKcs: implications for the therapy of human diseases.MedComm (2020). 2024 Jun 19;5(7):e613. doi: 10.1002/mco2.613. eCollection 2024 Jul. MedComm (2020). 2024. PMID: 38898995 Free PMC article. Review.
References
-
- Agrawal A., Schatz D.G. RAG1 and RAG2 form a stable postcleavage synaptic complex with DNA containing signal ends in V(D)J recombination. Cell. 1997;89:43–53. - PubMed
-
- Arnal S.M., Roth D.B. Excised V(D)J recombination byproducts threaten genomic integrity. Trends Immunol. 2007;28:289–292. - PubMed
-
- Bergeron S., Anderson D.K., Swanson P.C. RAG and HMGB1 Proteins: Purification and Biochemical Analysis of Recombination Signal Complexes. Methods Enzymol. 2006;408:511–528. - PubMed
-
- Bories J.C., Cayuela J.M., Loiseau P., Sigaux F. Expression of human recombination activating genes (RAG1 and RAG2) in neoplastic lymphoid cells: correlation with cell differentiation and antigen receptor expression. Blood. 1991;78:2053–2061. - PubMed
Publication types
MeSH terms
Substances
Grants and funding
LinkOut - more resources
Full Text Sources
Molecular Biology Databases
Research Materials
Miscellaneous