Nanotechnology for Neuroscience: Promising Approaches for Diagnostics, Therapeutics and Brain Activity Mapping
- PMID: 30853878
- PMCID: PMC6404766
- DOI: 10.1002/adfm.201700489
Nanotechnology for Neuroscience: Promising Approaches for Diagnostics, Therapeutics and Brain Activity Mapping
Abstract
Unlocking the secrets of the brain is a task fraught with complexity and challenge - not least due to the intricacy of the circuits involved. With advancements in the scale and precision of scientific technologies, we are increasingly equipped to explore how these components interact to produce a vast range of outputs that constitute function and disease. Here, an insight is offered into key areas in which the marriage of neuroscience and nanotechnology has revolutionized the industry. The evolution of ever more sophisticated nanomaterials culminates in network-operant functionalized agents. In turn, these materials contribute to novel diagnostic and therapeutic strategies, including drug delivery, neuroprotection, neural regeneration, neuroimaging and neurosurgery. Further, the entrance of nanotechnology into future research arenas including optogenetics, molecular/ion sensing and monitoring, and piezoelectric effects is discussed. Finally, considerations in nanoneurotoxicity, the main barrier to clinical translation, are reviewed, and direction for future perspectives is provided.
Keywords: brain activity mapping (BAM); nanoneuroscience; nanoneurotoxicity; neural regeneration; neuroimaging; optogenetics.
Conflict of interest statement
Conflict of Interest The authors declare no conflict of interest.
Figures
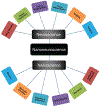
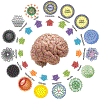
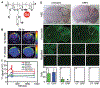
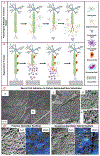
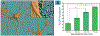
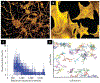
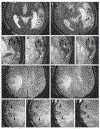
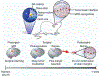
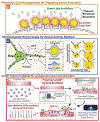
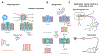
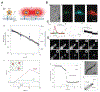
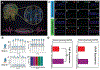
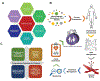
Similar articles
-
Neuro-nanotechnology: diagnostic and therapeutic nano-based strategies in applied neuroscience.Biomed Eng Online. 2023 Jan 2;22(1):1. doi: 10.1186/s12938-022-01062-y. Biomed Eng Online. 2023. PMID: 36593487 Free PMC article. Review.
-
Soft Bioelectronics Using Nanomaterials and Nanostructures for Neuroengineering.Acc Chem Res. 2024 Jun 4;57(11):1633-1647. doi: 10.1021/acs.accounts.4c00163. Epub 2024 May 16. Acc Chem Res. 2024. PMID: 38752397 Review.
-
Neurophotonics: a comprehensive review, current challenges and future trends.Front Neurosci. 2024 May 3;18:1382341. doi: 10.3389/fnins.2024.1382341. eCollection 2024. Front Neurosci. 2024. PMID: 38765670 Free PMC article. Review.
-
Programmable DNA Nanodevices for Applications in Neuroscience.ACS Chem Neurosci. 2021 Feb 3;12(3):363-377. doi: 10.1021/acschemneuro.0c00723. Epub 2021 Jan 12. ACS Chem Neurosci. 2021. PMID: 33433192
-
Multifunctional Nanocarriers for diagnostics, drug delivery and targeted treatment across blood-brain barrier: perspectives on tracking and neuroimaging.Part Fibre Toxicol. 2010 Mar 3;7:3. doi: 10.1186/1743-8977-7-3. Part Fibre Toxicol. 2010. PMID: 20199661 Free PMC article. Review.
Cited by
-
Polymeric nanoparticles for dopamine and levodopa replacement in Parkinson's disease.Nanoscale Adv. 2022 Nov 3;4(24):5233-5244. doi: 10.1039/d2na00524g. eCollection 2022 Dec 6. Nanoscale Adv. 2022. PMID: 36540116 Free PMC article. Review.
-
Neuro-nanotechnology: diagnostic and therapeutic nano-based strategies in applied neuroscience.Biomed Eng Online. 2023 Jan 2;22(1):1. doi: 10.1186/s12938-022-01062-y. Biomed Eng Online. 2023. PMID: 36593487 Free PMC article. Review.
-
Self-Assembly Protein Superstructures as a Powerful Chemodynamic Therapy Nanoagent for Glioblastoma Treatment.Nanomicro Lett. 2020 Jul 15;12(1):151. doi: 10.1007/s40820-020-00490-6. Nanomicro Lett. 2020. PMID: 34138164 Free PMC article.
-
Nanomaterial-assisted oncolytic bacteria in solid tumor diagnosis and therapeutics.Bioeng Transl Med. 2024 Apr 17;9(4):e10672. doi: 10.1002/btm2.10672. eCollection 2024 Jul. Bioeng Transl Med. 2024. PMID: 39036084 Free PMC article. Review.
-
Wearable sensors for monitoring marine environments and their inhabitants.Nat Biotechnol. 2023 Sep;41(9):1208-1220. doi: 10.1038/s41587-023-01827-3. Epub 2023 Jun 26. Nat Biotechnol. 2023. PMID: 37365259 Review.
References
-
- Bargmann CI, Marder E, Nat. Methods 2013, 10, 483. - PubMed
-
- Silva GA, Nat. Rev. Neurosci 2006, 7, 65. - PubMed
-
- Alivisatos AP, Andrews AM, Boyden ES, Chun M, Church GM, Deisseroth K, Donoghue JP, Fraser SE, Lippincott-Schwartz J, Looger LL, Masmanidis S, McEuen PL, Nurmikko AV, Park H, Peterka DS, Reid C, Roukes ML, Scherer A, Schnitzer M, Sejnowski TJ, Shepard KL, Tsao D, Turrigiano G, Weiss PS, Xu C, Yuste R, Zhuang X, ACS Nano 2013, 7, 1850. - PMC - PubMed
-
- Cabral J, Kringelbach ML, Deco G, Prog. Neurobiol 2014, 114, 102. - PubMed
Grants and funding
LinkOut - more resources
Full Text Sources
Miscellaneous