Calcium Sensors in Neuronal Function and Dysfunction
- PMID: 30833454
- PMCID: PMC6496351
- DOI: 10.1101/cshperspect.a035154
Calcium Sensors in Neuronal Function and Dysfunction
Abstract
Calcium signaling in neurons as in other cell types can lead to varied changes in cellular function. Neuronal Ca2+ signaling processes have also become adapted to modulate the function of specific pathways over a wide variety of time domains and these can have effects on, for example, axon outgrowth, neuronal survival, and changes in synaptic strength. Ca2+ also plays a key role in synapses as the trigger for fast neurotransmitter release. Given its physiological importance, abnormalities in neuronal Ca2+ signaling potentially underlie many different neurological and neurodegenerative diseases. The mechanisms by which changes in intracellular Ca2+ concentration in neurons can bring about diverse responses is underpinned by the roles of ubiquitous or specialized neuronal Ca2+ sensors. It has been established that synaptotagmins have key functions in neurotransmitter release, and, in addition to calmodulin, other families of EF-hand-containing neuronal Ca2+ sensors, including the neuronal calcium sensor (NCS) and the calcium-binding protein (CaBP) families, play important physiological roles in neuronal Ca2+ signaling. It has become increasingly apparent that these various Ca2+ sensors may also be crucial for aspects of neuronal dysfunction and disease either indirectly or directly as a direct consequence of genetic variation or mutations. An understanding of the molecular basis for the regulation of the targets of the Ca2+ sensors and the physiological roles of each protein in identified neurons may contribute to future approaches to the development of treatments for a variety of human neuronal disorders.
Copyright © 2019 Cold Spring Harbor Laboratory Press; all rights reserved.
Figures
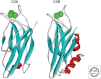
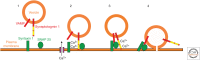
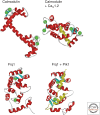
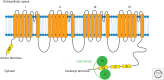
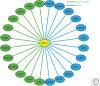
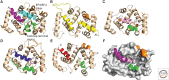
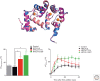
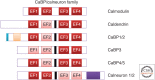
Similar articles
-
The diversity of calcium sensor proteins in the regulation of neuronal function.Cold Spring Harb Perspect Biol. 2010 Aug;2(8):a004085. doi: 10.1101/cshperspect.a004085. Epub 2010 Jul 28. Cold Spring Harb Perspect Biol. 2010. PMID: 20668007 Free PMC article. Review.
-
Sensing change: the emerging role of calcium sensors in neuronal disease.Semin Cell Dev Biol. 2011 Jul;22(5):530-5. doi: 10.1016/j.semcdb.2011.07.014. Epub 2011 Jul 22. Semin Cell Dev Biol. 2011. PMID: 21803168 Review.
-
The neuronal calcium-sensor proteins.Biochim Biophys Acta. 2004 Dec 6;1742(1-3):59-68. doi: 10.1016/j.bbamcr.2004.08.008. Biochim Biophys Acta. 2004. PMID: 15590056 Review.
-
Sense and specificity in neuronal calcium signalling.Biochim Biophys Acta. 2015 Sep;1853(9):1921-32. doi: 10.1016/j.bbamcr.2014.10.029. Epub 2014 Nov 4. Biochim Biophys Acta. 2015. PMID: 25447549 Free PMC article. Review.
-
Neuronal calcium sensor proteins: generating diversity in neuronal Ca2+ signalling.Nat Rev Neurosci. 2007 Mar;8(3):182-93. doi: 10.1038/nrn2093. Nat Rev Neurosci. 2007. PMID: 17311005 Free PMC article. Review.
Cited by
-
Sensing sound: Cellular specializations and molecular force sensors.Neuron. 2022 Nov 16;110(22):3667-3687. doi: 10.1016/j.neuron.2022.09.018. Epub 2022 Oct 11. Neuron. 2022. PMID: 36223766 Free PMC article. Review.
-
NCS1 overexpression restored mitochondrial activity and behavioral alterations in a zebrafish model of Wolfram syndrome.Mol Ther Methods Clin Dev. 2022 Oct 7;27:295-308. doi: 10.1016/j.omtm.2022.10.003. eCollection 2022 Dec 8. Mol Ther Methods Clin Dev. 2022. PMID: 36320410 Free PMC article.
-
Calcium Dyshomeostasis in Alzheimer's Disease Pathogenesis.Int J Mol Sci. 2021 May 6;22(9):4914. doi: 10.3390/ijms22094914. Int J Mol Sci. 2021. PMID: 34066371 Free PMC article. Review.
-
Prevention and Treatment Strategies for Alzheimer's Disease: Focusing on Microglia and Astrocytes in Neuroinflammation.J Inflamm Res. 2024 Oct 13;17:7235-7259. doi: 10.2147/JIR.S483412. eCollection 2024. J Inflamm Res. 2024. PMID: 39421566 Free PMC article. Review.
-
Cav2.3 channels contribute to dopaminergic neuron loss in a model of Parkinson's disease.Nat Commun. 2019 Nov 8;10(1):5094. doi: 10.1038/s41467-019-12834-x. Nat Commun. 2019. PMID: 31704946 Free PMC article.
References
-
- Alaimo A, Etxeberria A, Gómez-Posada JC, Gomis-Perez C, Fernandez-Orth J, Malo C, Villarroel A. 2018. Lack of correlation between surface expression and currents in epileptogenic AB-calmodulin binding domain Kv7.2 potassium channel mutants. Channels (Austin) 12: 299–310. 10.1080/19336950.2018.1511512 - DOI - PMC - PubMed
Publication types
MeSH terms
Substances
Grants and funding
LinkOut - more resources
Full Text Sources
Research Materials
Miscellaneous