A Genome-Wide Epstein-Barr Virus Polyadenylation Map and Its Antisense RNA to EBNA
- PMID: 30355690
- PMCID: PMC6321932
- DOI: 10.1128/JVI.01593-18
A Genome-Wide Epstein-Barr Virus Polyadenylation Map and Its Antisense RNA to EBNA
Abstract
Epstein-Barr virus (EBV) is a ubiquitous human pathogen associated with Burkitt's lymphoma and nasopharyngeal carcinoma. Although the EBV genome harbors more than a hundred genes, a full transcription map with EBV polyadenylation profiles remains unknown. To elucidate the 3' ends of all EBV transcripts genome-wide, we performed the first comprehensive analysis of viral polyadenylation sites (pA sites) using our previously reported polyadenylation sequencing (PA-seq) technology. We identified that EBV utilizes a total of 62 pA sites in JSC-1, 60 in Raji, and 53 in Akata cells for the expression of EBV genes from both plus and minus DNA strands; 42 of these pA sites are commonly used in all three cell lines. The majority of identified pA sites were mapped to the intergenic regions downstream of previously annotated EBV open reading frames (ORFs) and viral promoters. pA sites lacking an association with any known EBV genes were also identified, mostly for the minus DNA strand within the EBNA locus, a major locus responsible for maintenance of viral latency and cell transformation. The expression of these novel antisense transcripts to EBNA were verified by 3' rapid amplification of cDNA ends (RACE) and Northern blot analyses in several EBV-positive (EBV+) cell lines. In contrast to EBNA RNA expressed during latency, expression of EBNA-antisense transcripts, which is restricted in latent cells, can be significantly induced by viral lytic infection, suggesting potential regulation of viral gene expression by EBNA-antisense transcription during lytic EBV infection. Our data provide the first evidence that EBV has an unrecognized mechanism that regulates EBV reactivation from latency.IMPORTANCE Epstein-Barr virus represents an important human pathogen with an etiological role in the development of several cancers. By elucidation of a genome-wide polyadenylation landscape of EBV in JSC-1, Raji, and Akata cells, we have redefined the EBV transcriptome and mapped individual polymerase II (Pol II) transcripts of viral genes to each one of the mapped pA sites at single-nucleotide resolution as well as the depth of expression. By unveiling a new class of viral lytic RNA transcripts antisense to latent EBNAs, we provide a novel mechanism of how EBV might control the expression of viral latent genes and lytic infection. Thus, this report takes another step closer to understanding EBV gene structure and expression and paves a new path for antiviral approaches.
Keywords: Epstein-Barr virus; RNA-seq; antisense RNA; genome-wide approach; polyadenylation site mapping; tumor virus.
This is a work of the U.S. Government and is not subject to copyright protection in the United States. Foreign copyrights may apply.
Figures
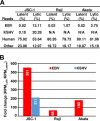
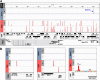
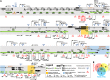
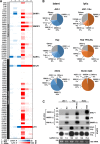
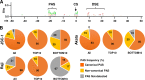
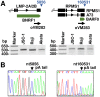
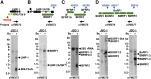
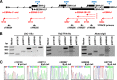
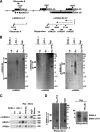
Similar articles
-
Reactivation of Epstein-Barr Virus from Latency Involves Increased RNA Polymerase Activity at CTCF Binding Sites on the Viral Genome.J Virol. 2023 Feb 28;97(2):e0189422. doi: 10.1128/jvi.01894-22. Epub 2023 Feb 6. J Virol. 2023. PMID: 36744959 Free PMC article.
-
RNA Sequencing Analyses of Gene Expression during Epstein-Barr Virus Infection of Primary B Lymphocytes.J Virol. 2019 Jun 14;93(13):e00226-19. doi: 10.1128/JVI.00226-19. Print 2019 Jul 1. J Virol. 2019. PMID: 31019051 Free PMC article.
-
Autorepression of Epstein-Barr virus nuclear antigen 1 expression by inhibition of pre-mRNA processing.J Virol. 2008 Feb;82(4):1679-87. doi: 10.1128/JVI.02142-07. Epub 2007 Dec 12. J Virol. 2008. PMID: 18077719 Free PMC article.
-
Epstein-Barr virus latent genes.Exp Mol Med. 2015 Jan 23;47(1):e131. doi: 10.1038/emm.2014.84. Exp Mol Med. 2015. PMID: 25613728 Free PMC article. Review.
-
Epigenetic regulation of latent Epstein-Barr virus promoters.Biochim Biophys Acta. 2010 Mar-Apr;1799(3-4):228-35. doi: 10.1016/j.bbagrm.2009.10.005. Epub 2009 Oct 22. Biochim Biophys Acta. 2010. PMID: 19853674 Review.
Cited by
-
Circ-Udg Derived from Cyprinid Herpesvirus 2 Promotes Viral Replication.Microbiol Spectr. 2022 Aug 31;10(4):e0094322. doi: 10.1128/spectrum.00943-22. Epub 2022 Jun 30. Microbiol Spectr. 2022. PMID: 35770986 Free PMC article.
-
Epstein-Barr Virus and Multiple Sclerosis.Front Immunol. 2020 Dec 17;11:587078. doi: 10.3389/fimmu.2020.587078. eCollection 2020. Front Immunol. 2020. PMID: 33391262 Free PMC article. Review.
-
Comprehensive Investigations Relationship Between Viral Infections and Multiple Sclerosis Pathogenesis.Curr Microbiol. 2022 Dec 2;80(1):15. doi: 10.1007/s00284-022-03112-z. Curr Microbiol. 2022. PMID: 36459252 Free PMC article. Review.
-
Novel EBV LMP-2-affibody and affitoxin in molecular imaging and targeted therapy of nasopharyngeal carcinoma.PLoS Pathog. 2020 Jan 6;16(1):e1008223. doi: 10.1371/journal.ppat.1008223. eCollection 2020 Jan. PLoS Pathog. 2020. PMID: 31905218 Free PMC article.
-
Integrative profiling of Epstein-Barr virus transcriptome using a multiplatform approach.Virol J. 2022 Jan 6;19(1):7. doi: 10.1186/s12985-021-01734-6. Virol J. 2022. PMID: 34991630 Free PMC article.
References
-
- Bass AJ, Thorsson V, Shmulevich I, Reynolds SM, Miller M, Bernard B, Hinoue T, Laird PW, Curtis C, Shen H, Weisenberger DJ, Schultz N, Shen R, Weinhold N, Kelsen DP, Bowlby R, Chu A, Kasaian K, Mungall AJ, Gordon Robertson A, Sipahimalani P, Cherniack A, Getz G, Liu Y, Noble MS, Pedamallu C, Sougnez C, Taylor-Weiner A, Akbani R, Lee J-S, Liu W, Mills GB, Yang D, Zhang W, Pantazi A, Parfenov M, Gulley M, Blanca Piazuelo M, Schneider BG, Kim J, Boussioutas A, Sheth M, Demchok JA, Rabkin CS, Willis JE, Ng S, Garman K, Beer DG, Pennathur A, Raphael BJ, et al. 2014. Comprehensive molecular characterization of gastric adenocarcinoma. Nature 513:202–209. doi:10.1038/nature13480. - DOI - PMC - PubMed
Publication types
MeSH terms
Substances
Grants and funding
LinkOut - more resources
Full Text Sources