Phase transitions as intermediate steps in the formation of molecularly engineered protein fibers
- PMID: 30271967
- PMCID: PMC6123624
- DOI: 10.1038/s42003-018-0090-y
Phase transitions as intermediate steps in the formation of molecularly engineered protein fibers
Abstract
A central concept in molecular bioscience is how structure formation at different length scales is achieved. Here we use spider silk protein as a model to design new recombinant proteins that assemble into fibers. We made proteins with a three-block architecture with folded globular domains at each terminus of a truncated repetitive silk sequence. Aqueous solutions of these engineered proteins undergo liquid-liquid phase separation as an essential pre-assembly step before fibers can form by drawing in air. We show that two different forms of phase separation occur depending on solution conditions, but only one form leads to fiber assembly. Structural variants with one-block or two-block architectures do not lead to fibers. Fibers show strong adhesion to surfaces and self-fusing properties when placed into contact with each other. Our results show a link between protein architecture and phase separation behavior suggesting a general approach for understanding protein assembly from dilute solutions into functional structures.
Conflict of interest statement
The authors declare no competing interests.
Figures
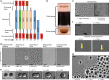
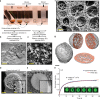
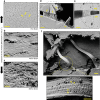
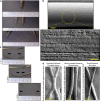
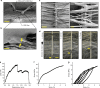
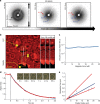
Similar articles
-
Self-Coacervation of a Silk-Like Protein and Its Use As an Adhesive for Cellulosic Materials.ACS Macro Lett. 2018 Sep 18;7(9):1120-1125. doi: 10.1021/acsmacrolett.8b00527. Epub 2018 Sep 5. ACS Macro Lett. 2018. PMID: 30258700 Free PMC article.
-
Spider silk self-assembly via modular liquid-liquid phase separation and nanofibrillation.Sci Adv. 2020 Nov 4;6(45):eabb6030. doi: 10.1126/sciadv.abb6030. Print 2020 Nov. Sci Adv. 2020. PMID: 33148640 Free PMC article.
-
Liquid-Liquid Phase Separation and Assembly of Silk-like Proteins is Dependent on the Polymer Length.Biomacromolecules. 2022 Aug 8;23(8):3142-3153. doi: 10.1021/acs.biomac.2c00179. Epub 2022 Jul 7. Biomacromolecules. 2022. PMID: 35796676 Free PMC article.
-
Review the role of terminal domains during storage and assembly of spider silk proteins.Biopolymers. 2012 Jun;97(6):355-61. doi: 10.1002/bip.22006. Epub 2011 Nov 5. Biopolymers. 2012. PMID: 22057429 Review.
-
A structural view on spider silk proteins and their role in fiber assembly.J Pept Sci. 2012 Jun;18(6):357-65. doi: 10.1002/psc.2417. Epub 2012 May 8. J Pept Sci. 2012. PMID: 22570231 Review.
Cited by
-
Metal ions guide the production of silkworm silk fibers.Nat Commun. 2024 Aug 6;15(1):6671. doi: 10.1038/s41467-024-50879-9. Nat Commun. 2024. PMID: 39107276 Free PMC article.
-
Self-Coacervation of a Silk-Like Protein and Its Use As an Adhesive for Cellulosic Materials.ACS Macro Lett. 2018 Sep 18;7(9):1120-1125. doi: 10.1021/acsmacrolett.8b00527. Epub 2018 Sep 5. ACS Macro Lett. 2018. PMID: 30258700 Free PMC article.
-
The mechanism driving a solid-solid phase transition in a biomacromolecular crystal.IUCrJ. 2021 Jun 17;8(Pt 4):655-664. doi: 10.1107/S2052252521004826. eCollection 2021 Jul 1. IUCrJ. 2021. PMID: 34258013 Free PMC article.
-
Spider silk self-assembly via modular liquid-liquid phase separation and nanofibrillation.Sci Adv. 2020 Nov 4;6(45):eabb6030. doi: 10.1126/sciadv.abb6030. Print 2020 Nov. Sci Adv. 2020. PMID: 33148640 Free PMC article.
-
Recombinant protein condensation inside E. coli enables the development of building blocks for bioinspired materials engineering - Biomimetic spider silk protein as a case study.Mater Today Bio. 2022 Nov 14;17:100492. doi: 10.1016/j.mtbio.2022.100492. eCollection 2022 Dec 15. Mater Today Bio. 2022. PMID: 36420055 Free PMC article.
References
LinkOut - more resources
Full Text Sources
Other Literature Sources