Visualizing and discovering cellular structures with super-resolution microscopy
- PMID: 30166485
- PMCID: PMC6535400
- DOI: 10.1126/science.aau1044
Visualizing and discovering cellular structures with super-resolution microscopy
Abstract
Super-resolution microscopy has overcome a long-held resolution barrier-the diffraction limit-in light microscopy and enabled visualization of previously invisible molecular details in biological systems. Since their conception, super-resolution imaging methods have continually evolved and can now be used to image cellular structures in three dimensions, multiple colors, and living systems with nanometer-scale resolution. These methods have been applied to answer questions involving the organization, interaction, stoichiometry, and dynamics of individual molecular building blocks and their integration into functional machineries in cells and tissues. In this Review, we provide an overview of super-resolution methods, their state-of-the-art capabilities, and their constantly expanding applications to biology, with a focus on the latter. We will also describe the current technical challenges and future advances anticipated in super-resolution imaging.
Copyright © 2018, American Association for the Advancement of Science.
Conflict of interest statement
Figures
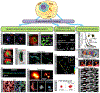
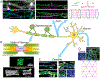
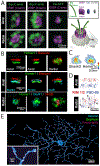
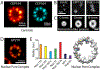
Similar articles
-
Super-resolution fluorescence microscopy.Annu Rev Biochem. 2009;78:993-1016. doi: 10.1146/annurev.biochem.77.061906.092014. Annu Rev Biochem. 2009. PMID: 19489737 Free PMC article. Review.
-
Advances in light-based imaging of three-dimensional cellular ultrastructure.Curr Opin Cell Biol. 2012 Feb;24(1):125-33. doi: 10.1016/j.ceb.2011.11.010. Epub 2011 Dec 30. Curr Opin Cell Biol. 2012. PMID: 22209239 Free PMC article. Review.
-
Cell biology of the future: Nanometer-scale cellular cartography.J Cell Biol. 2015 Oct 26;211(2):211-4. doi: 10.1083/jcb.201508021. Epub 2015 Oct 19. J Cell Biol. 2015. PMID: 26483557 Free PMC article.
-
[Comparison and progress review of various super-resolution fluorescence imaging techniques].Se Pu. 2021 Oct;39(10):1055-1064. doi: 10.3724/SP.J.1123.2021.06015. Se Pu. 2021. PMID: 34505427 Free PMC article. Review. Chinese.
-
Super-resolution fluorescence imaging with single molecules.Curr Opin Struct Biol. 2013 Oct;23(5):778-87. doi: 10.1016/j.sbi.2013.07.010. Epub 2013 Aug 8. Curr Opin Struct Biol. 2013. PMID: 23932284 Free PMC article. Review.
Cited by
-
STochastic Optical Reconstruction Microscopy (STORM) reveals the nanoscale organization of pathological aggregates in human brain.Neuropathol Appl Neurobiol. 2021 Feb;47(1):127-142. doi: 10.1111/nan.12646. Epub 2020 Aug 12. Neuropathol Appl Neurobiol. 2021. PMID: 32688444 Free PMC article.
-
Optical super-resolution nanothermometry via stimulated emission depletion imaging of upconverting nanoparticles.Sci Adv. 2024 Jul 19;10(29):eado6268. doi: 10.1126/sciadv.ado6268. Epub 2024 Jul 17. Sci Adv. 2024. PMID: 39018395 Free PMC article.
-
Quantum Dots for Improved Single-Molecule Localization Microscopy.J Phys Chem B. 2021 Mar 18;125(10):2566-2576. doi: 10.1021/acs.jpcb.0c11545. Epub 2021 Mar 8. J Phys Chem B. 2021. PMID: 33683893 Free PMC article.
-
Super-resolved live-cell imaging using random illumination microscopy.Cell Rep Methods. 2021 Apr 30;1(1):100009. doi: 10.1016/j.crmeth.2021.100009. eCollection 2021 May 24. Cell Rep Methods. 2021. PMID: 35474693 Free PMC article.
-
The Mechanisms of Thin Filament Assembly and Length Regulation in Muscles.Int J Mol Sci. 2022 May 10;23(10):5306. doi: 10.3390/ijms23105306. Int J Mol Sci. 2022. PMID: 35628117 Free PMC article. Review.
References
-
- Hell SW, Wichmann J, Breaking the diffraction resolution limit by stimulated emission: stimulated-emission-depletion fluorescence microscopy. Optics letters 19, 780–782 (1994). - PubMed
-
- Klar TA, Hell SW, Subdiffraction resolution in far-field fluorescence microscopy. Optics letters 24, 954–956 (1999). - PubMed
-
- Eggeling C, Willig KI, Sahl SJ, Hell SW, Lens-based fluorescence nanoscopy. Quarterly reviews of biophysics 48, 178–243 (2015). - PubMed
-
- Heintzmann R, Gustafsson MGL, Subdiffraction resolution in continuous samples. Nature Photonics 3, 362–364 (2009).
Publication types
MeSH terms
Grants and funding
LinkOut - more resources
Full Text Sources
Other Literature Sources