The temperature dependence of the helical twist of DNA
- PMID: 30053087
- PMCID: PMC6125625
- DOI: 10.1093/nar/gky599
The temperature dependence of the helical twist of DNA
Abstract
DNA is the carrier of all cellular genetic information and increasingly used in nanotechnology. Quantitative understanding and optimization of its functions requires precise experimental characterization and accurate modeling of DNA properties. A defining feature of DNA is its helicity. DNA unwinds with increasing temperature, even for temperatures well below the melting temperature. However, accurate quantitation of DNA unwinding under external forces and a microscopic understanding of the corresponding structural changes are currently lacking. Here we combine single-molecule magnetic tweezers measurements with atomistic molecular dynamics and coarse-grained simulations to obtain a comprehensive view of the temperature dependence of DNA twist. Experimentally, we find that DNA twist changes by ΔTw(T) = (-11.0 ± 1.2)°/(°C·kbp), independent of applied force, in the range of forces where torque-induced melting is negligible. Our atomistic simulations predict ΔTw(T) = (-11.1 ± 0.3)°/(°C·kbp), in quantitative agreement with experiments, and suggest that the untwisting of DNA with temperature is predominantly due to changes in DNA structure for defined backbone substates, while the effects of changes in substate populations are minor. Coarse-grained simulations using the oxDNA framework yield a value of ΔTw(T) = (-6.4 ± 0.2)°/(°C·kbp) in semi-quantitative agreement with experiments.
Figures
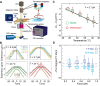
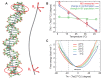
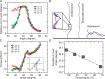
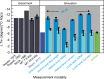
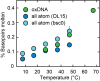
Similar articles
-
Temperature-Dependent Twist of Double-Stranded RNA Probed by Magnetic Tweezer Experiments and Molecular Dynamics Simulations.J Phys Chem B. 2024 Jan 25;128(3):664-675. doi: 10.1021/acs.jpcb.3c06280. Epub 2024 Jan 10. J Phys Chem B. 2024. PMID: 38197365 Free PMC article.
-
Simulations of DNA denaturation dynamics under constrained conditions.J Phys Condens Matter. 2022 May 24;34(29). doi: 10.1088/1361-648X/ac6d39. J Phys Condens Matter. 2022. PMID: 35512678
-
Temperature dependence of DNA elasticity: An all-atom molecular dynamics simulation study.J Chem Phys. 2023 Mar 7;158(9):094902. doi: 10.1063/5.0138940. J Chem Phys. 2023. PMID: 36889965
-
Probing the mechanical properties, conformational changes, and interactions of nucleic acids with magnetic tweezers.J Struct Biol. 2017 Jan;197(1):26-36. doi: 10.1016/j.jsb.2016.06.022. Epub 2016 Jun 29. J Struct Biol. 2017. PMID: 27368129 Review.
-
A Primer on the oxDNA Model of DNA: When to Use it, How to Simulate it and How to Interpret the Results.Front Mol Biosci. 2021 Jun 17;8:693710. doi: 10.3389/fmolb.2021.693710. eCollection 2021. Front Mol Biosci. 2021. PMID: 34235181 Free PMC article. Review.
Cited by
-
Suitability of double-stranded DNA as a molecular standard for the validation of analytical ultracentrifugation instruments.Eur Biophys J. 2023 Jul;52(4-5):267-280. doi: 10.1007/s00249-023-01671-y. Epub 2023 Jul 27. Eur Biophys J. 2023. PMID: 37501021 Free PMC article.
-
Temperature-dependent elasticity of DNA, RNA, and hybrid double helices.Biophys J. 2024 Mar 5;123(5):572-583. doi: 10.1016/j.bpj.2024.01.032. Epub 2024 Feb 2. Biophys J. 2024. PMID: 38340722 Free PMC article.
-
RNA Captures More Cations than DNA: Insights from Molecular Dynamics Simulations.J Phys Chem B. 2022 Nov 3;126(43):8646-8654. doi: 10.1021/acs.jpcb.2c04488. Epub 2022 Oct 19. J Phys Chem B. 2022. PMID: 36260822 Free PMC article.
-
Effect of temperature on anisotropic bending elasticity of dsRNA: an all-atom molecular dynamics simulation.RSC Adv. 2024 May 28;14(24):17170-17177. doi: 10.1039/d4ra02354d. eCollection 2024 May 22. RSC Adv. 2024. PMID: 38808231 Free PMC article.
-
Twist-diameter coupling drives DNA twist changes with salt and temperature.Sci Adv. 2022 Mar 25;8(12):eabn1384. doi: 10.1126/sciadv.abn1384. Epub 2022 Mar 23. Sci Adv. 2022. PMID: 35319990 Free PMC article.
References
-
- Strick T., Allemand J., Croquette V., Bensimon D.. Twisting and stretching single DNA molecules. Prog. Biophys. Mol. Biol. 2000; 74:115–140. - PubMed
-
- Bustamante C., Bryant Z., Smith S.B.. Ten years of tension: single-molecule DNA mechanics. Nature. 2003; 421:423–427. - PubMed
-
- Kriegel F., Ermann N., Lipfert J.. Probing the mechanical properties, conformational changes, and interactions of nucleic acids with magnetic tweezers. J. Struct. Biol. 2017; 197:26–36. - PubMed
-
- Srinivasan J., Cheatham T.E., Cieplak P.. Continuum solvent studies of the stability of DNA, RNA, and phosphoramidate− DNA helices. J. Am. Chem. Soc. 1998; 120:9401–9409.
Publication types
MeSH terms
Substances
LinkOut - more resources
Full Text Sources
Other Literature Sources