Conformational flexibility within the nascent polypeptide-associated complex enables its interactions with structurally diverse client proteins
- PMID: 29650757
- PMCID: PMC5986199
- DOI: 10.1074/jbc.RA117.001568
Conformational flexibility within the nascent polypeptide-associated complex enables its interactions with structurally diverse client proteins
Abstract
As newly synthesized polypeptides emerge from the ribosome, it is crucial that they fold correctly. To prevent premature aggregation, nascent chains interact with chaperones that facilitate folding or prevent misfolding until protein synthesis is complete. Nascent polypeptide-associated complex (NAC) is a ribosome-associated chaperone that is important for protein homeostasis. However, how NAC binds its substrates remains unclear. Using native electrospray ionization MS (ESI-MS), limited proteolysis, NMR, and cross-linking, we analyzed the conformational properties of NAC from Caenorhabditis elegans and studied its ability to bind proteins in different conformational states. Our results revealed that NAC adopts an array of compact and expanded conformations and binds weakly to client proteins that are unfolded, folded, or intrinsically disordered, suggestive of broad substrate compatibility. Of note, we found that this weak binding retards aggregation of the intrinsically disordered protein α-synuclein both in vitro and in vivo These findings provide critical insights into the structure and function of NAC. Specifically, they reveal the ability of NAC to exploit its conformational plasticity to bind a repertoire of substrates with unrelated sequences and structures, independently of actively translating ribosomes.
Keywords: NAC native mass spectrometry (MS); chaperone; molecular chaperone; nuclear magnetic resonance (NMR); protein cross-linking; protein folding; protein misfolding.
© 2018 by The American Society for Biochemistry and Molecular Biology, Inc.
Conflict of interest statement
The authors declare that they have no conflicts of interest with the contents of this article
Figures
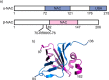
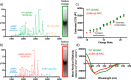
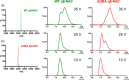
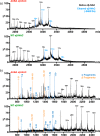
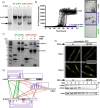
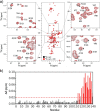
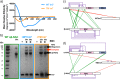
Similar articles
-
The nascent polypeptide-associated complex is a key regulator of proteostasis.EMBO J. 2013 May 15;32(10):1451-68. doi: 10.1038/emboj.2013.87. Epub 2013 Apr 19. EMBO J. 2013. PMID: 23604074 Free PMC article.
-
Defining the specificity of cotranslationally acting chaperones by systematic analysis of mRNAs associated with ribosome-nascent chain complexes.PLoS Biol. 2011 Jul;9(7):e1001100. doi: 10.1371/journal.pbio.1001100. Epub 2011 Jul 12. PLoS Biol. 2011. PMID: 21765803 Free PMC article.
-
The nascent polypeptide-associated complex is essential for autophagic flux.Autophagy. 2014 Oct 1;10(10):1738-48. doi: 10.4161/auto.29638. Epub 2014 Jul 18. Autophagy. 2014. PMID: 25126725 Free PMC article.
-
Molecular guardians for newborn proteins: ribosome-associated chaperones and their role in protein folding.Cell Mol Life Sci. 2005 Dec;62(23):2727-38. doi: 10.1007/s00018-005-5292-z. Cell Mol Life Sci. 2005. PMID: 16231086 Free PMC article. Review.
-
How Does the Ribosome Fold the Proteome?Annu Rev Biochem. 2020 Jun 20;89:389-415. doi: 10.1146/annurev-biochem-062917-012226. Annu Rev Biochem. 2020. PMID: 32569518 Review.
Cited by
-
Chaperone Interactions at the Ribosome.Cold Spring Harb Perspect Biol. 2019 Nov 1;11(11):a033977. doi: 10.1101/cshperspect.a033977. Cold Spring Harb Perspect Biol. 2019. PMID: 30833456 Free PMC article. Review.
-
The beta Subunit of Nascent Polypeptide Associated Complex Plays A Role in Flowers and Siliques Development of Arabidopsis thaliana.Int J Mol Sci. 2020 Mar 17;21(6):2065. doi: 10.3390/ijms21062065. Int J Mol Sci. 2020. PMID: 32192231 Free PMC article.
-
Structural Proteomics Methods to Interrogate the Conformations and Dynamics of Intrinsically Disordered Proteins.Front Chem. 2021 Mar 11;9:603639. doi: 10.3389/fchem.2021.603639. eCollection 2021. Front Chem. 2021. PMID: 33791275 Free PMC article. Review.
-
The nascent polypeptide-associated complex (NAC) controls translation initiation in cis by recruiting nucleolin to the encoding mRNA.Nucleic Acids Res. 2022 Sep 23;50(17):10110-10122. doi: 10.1093/nar/gkac751. Nucleic Acids Res. 2022. PMID: 36107769 Free PMC article.
-
The ribosomal chaperone NACA recruits PHD2 to cotranslationally modify HIF-α.EMBO J. 2022 Nov 17;41(22):e112059. doi: 10.15252/embj.2022112059. Epub 2022 Oct 11. EMBO J. 2022. PMID: 36219563 Free PMC article.
References
Publication types
MeSH terms
Substances
Associated data
- Actions
Grants and funding
- R01 GM102829/GM/NIGMS NIH HHS/United States
- BB/E012558/1/BB_/Biotechnology and Biological Sciences Research Council/United Kingdom
- BB/M012573/1/BB_/Biotechnology and Biological Sciences Research Council/United Kingdom
- 094232/WT_/Wellcome Trust/United Kingdom
- 089311/Z/09/Z/WT_/Wellcome Trust/United Kingdom
LinkOut - more resources
Full Text Sources
Other Literature Sources
Research Materials