Single-molecule visualization of Saccharomyces cerevisiae leading-strand synthesis reveals dynamic interaction between MTC and the replisome
- PMID: 28923950
- PMCID: PMC5635917
- DOI: 10.1073/pnas.1711291114
Single-molecule visualization of Saccharomyces cerevisiae leading-strand synthesis reveals dynamic interaction between MTC and the replisome
Abstract
The replisome, the multiprotein system responsible for genome duplication, is a highly dynamic complex displaying a large number of different enzyme activities. Recently, the Saccharomyces cerevisiae minimal replication reaction has been successfully reconstituted in vitro. This provided an opportunity to uncover the enzymatic activities of many of the components in a eukaryotic system. Their dynamic behavior and interactions in the context of the replisome, however, remain unclear. We use a tethered-bead assay to provide real-time visualization of leading-strand synthesis by the S. cerevisiae replisome at the single-molecule level. The minimal reconstituted leading-strand replisome requires 24 proteins, forming the CMG helicase, the Pol ε DNA polymerase, the RFC clamp loader, the PCNA sliding clamp, and the RPA single-stranded DNA binding protein. We observe rates and product lengths similar to those obtained from ensemble biochemical experiments. At the single-molecule level, we probe the behavior of two components of the replication progression complex and characterize their interaction with active leading-strand replisomes. The Minichromosome maintenance protein 10 (Mcm10), an important player in CMG activation, increases the number of productive replication events in our assay. Furthermore, we show that the fork protection complex Mrc1-Tof1-Csm3 (MTC) enhances the rate of the leading-strand replisome threefold. The introduction of periods of fast replication by MTC leads to an average rate enhancement of a factor of 2, similar to observations in cellular studies. We observe that the MTC complex acts in a dynamic fashion with the moving replisome, leading to alternating phases of slow and fast replication.
Keywords: CMG; DNA replication; Mrc1; replisome; single-molecule biophysics.
Conflict of interest statement
The authors declare no conflict of interest.
Figures
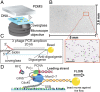
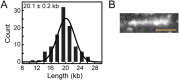
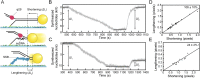
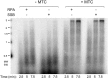
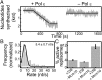
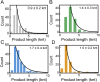
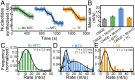
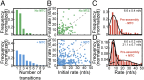
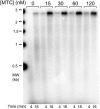
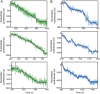
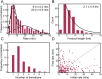
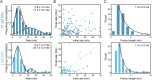
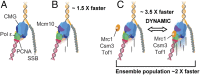
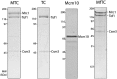
Similar articles
-
Cryo-EM Structure of the Fork Protection Complex Bound to CMG at a Replication Fork.Mol Cell. 2020 Jun 4;78(5):926-940.e13. doi: 10.1016/j.molcel.2020.04.012. Epub 2020 May 4. Mol Cell. 2020. PMID: 32369734 Free PMC article.
-
How the Eukaryotic Replisome Achieves Rapid and Efficient DNA Replication.Mol Cell. 2017 Jan 5;65(1):105-116. doi: 10.1016/j.molcel.2016.11.017. Epub 2016 Dec 15. Mol Cell. 2017. PMID: 27989442 Free PMC article.
-
Quality control mechanisms exclude incorrect polymerases from the eukaryotic replication fork.Proc Natl Acad Sci U S A. 2017 Jan 24;114(4):675-680. doi: 10.1073/pnas.1619748114. Epub 2017 Jan 9. Proc Natl Acad Sci U S A. 2017. PMID: 28069954 Free PMC article.
-
New Insights into the Mechanism of DNA Duplication by the Eukaryotic Replisome.Trends Biochem Sci. 2016 Oct;41(10):859-871. doi: 10.1016/j.tibs.2016.07.011. Epub 2016 Aug 20. Trends Biochem Sci. 2016. PMID: 27555051 Review.
-
Architecture of the Saccharomyces cerevisiae Replisome.Adv Exp Med Biol. 2017;1042:207-228. doi: 10.1007/978-981-10-6955-0_10. Adv Exp Med Biol. 2017. PMID: 29357060 Free PMC article. Review.
Cited by
-
Duplex DNA engagement and RPA oppositely regulate the DNA-unwinding rate of CMG helicase.Nat Commun. 2020 Jul 24;11(1):3713. doi: 10.1038/s41467-020-17443-7. Nat Commun. 2020. PMID: 32709841 Free PMC article.
-
Visualizing the dynamics of DNA replication and repair at the single-molecule level.Methods Cell Biol. 2024;182:109-165. doi: 10.1016/bs.mcb.2023.07.001. Epub 2023 Aug 10. Methods Cell Biol. 2024. PMID: 38359974 Free PMC article.
-
Dynamics of the Eukaryotic Replicative Helicase at Lagging-Strand Protein Barriers Support the Steric Exclusion Model.Cell Rep. 2019 Feb 19;26(8):2113-2125.e6. doi: 10.1016/j.celrep.2019.01.086. Cell Rep. 2019. PMID: 30784593 Free PMC article.
-
RecF protein targeting to post-replication (daughter strand) gaps II: RecF interaction with replisomes.Nucleic Acids Res. 2023 Jun 23;51(11):5714-5742. doi: 10.1093/nar/gkad310. Nucleic Acids Res. 2023. PMID: 37125644 Free PMC article.
-
Molecular Basis for ATP-Hydrolysis-Driven DNA Translocation by the CMG Helicase of the Eukaryotic Replisome.Cell Rep. 2019 Sep 3;28(10):2673-2688.e8. doi: 10.1016/j.celrep.2019.07.104. Cell Rep. 2019. PMID: 31484077 Free PMC article.
References
Publication types
MeSH terms
Substances
Grants and funding
LinkOut - more resources
Full Text Sources
Other Literature Sources
Molecular Biology Databases
Miscellaneous