Mechanical signals activate p38 MAPK pathway-dependent reinforcement of actin via mechanosensitive HspB1
- PMID: 28768826
- PMCID: PMC5620374
- DOI: 10.1091/mbc.E17-02-0087
Mechanical signals activate p38 MAPK pathway-dependent reinforcement of actin via mechanosensitive HspB1
Abstract
Despite the importance of a cell's ability to sense and respond to mechanical force, the molecular mechanisms by which physical cues are converted to cell-instructive chemical information to influence cell behaviors remain to be elucidated. Exposure of cultured fibroblasts to uniaxial cyclic stretch results in an actin stress fiber reinforcement response that stabilizes the actin cytoskeleton. p38 MAPK signaling is activated in response to stretch, and inhibition of p38 MAPK abrogates stretch-induced cytoskeletal reorganization. Here we show that the small heat shock protein HspB1 (hsp25/27) is phosphorylated in stretch-stimulated mouse fibroblasts via a p38 MAPK-dependent mechanism. Phosphorylated HspB1 is recruited to the actin cytoskeleton, displaying prominent accumulation on actin "comet tails" that emanate from focal adhesions in stretch-stimulated cells. Site-directed mutagenesis to block HspB1 phosphorylation inhibits the protein's cytoskeletal recruitment in response to mechanical stimulation. HspB1-null cells, generated by CRISPR/Cas9 nuclease genome editing, display an abrogated stretch-stimulated actin reinforcement response and increased cell migration. HspB1 is recruited to sites of increased traction force in cells geometrically constrained on micropatterned substrates. Our findings elucidate a molecular pathway by which a mechanical signal is transduced via activation of p38 MAPK to influence actin remodeling and cell migration via a zyxin-independent process.
© 2017 Hoffman et al. This article is distributed by The American Society for Cell Biology under license from the author(s). Two months after publication it is available to the public under an Attribution–Noncommercial–Share Alike 3.0 Unported Creative Commons License (http://creativecommons.org/licenses/by-nc-sa/3.0).
Figures
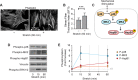
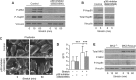
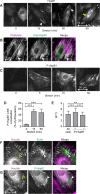
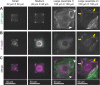
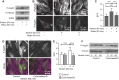
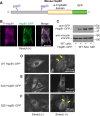
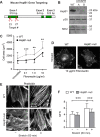
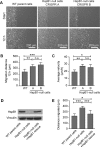
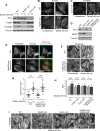
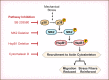
Similar articles
-
Phosphorylation of the small heat shock protein HspB1 regulates cytoskeletal recruitment and cell motility.Mol Biol Cell. 2022 Sep 15;33(11):ar100. doi: 10.1091/mbc.E22-02-0057. Epub 2022 Jun 29. Mol Biol Cell. 2022. PMID: 35767320 Free PMC article.
-
Mechanical force mobilizes zyxin from focal adhesions to actin filaments and regulates cytoskeletal reinforcement.J Cell Biol. 2005 Oct 24;171(2):209-15. doi: 10.1083/jcb.200505018. J Cell Biol. 2005. PMID: 16247023 Free PMC article.
-
Stretch-induced actin remodeling requires targeting of zyxin to stress fibers and recruitment of actin regulators.Mol Biol Cell. 2012 May;23(10):1846-59. doi: 10.1091/mbc.E11-12-1057. Epub 2012 Mar 28. Mol Biol Cell. 2012. PMID: 22456508 Free PMC article.
-
The actin cytoskeleton response to oxidants: from small heat shock protein phosphorylation to changes in the redox state of actin itself.Free Radic Biol Med. 2001 Dec 15;31(12):1624-32. doi: 10.1016/s0891-5849(01)00749-3. Free Radic Biol Med. 2001. PMID: 11744337 Review.
-
Modulation of actin dynamics during stress and physiological stimulation by a signaling pathway involving p38 MAP kinase and heat-shock protein 27.Biochem Cell Biol. 1995 Sep-Oct;73(9-10):703-7. doi: 10.1139/o95-078. Biochem Cell Biol. 1995. PMID: 8714691 Review.
Cited by
-
Phosphorylation of the small heat shock protein HspB1 regulates cytoskeletal recruitment and cell motility.Mol Biol Cell. 2022 Sep 15;33(11):ar100. doi: 10.1091/mbc.E22-02-0057. Epub 2022 Jun 29. Mol Biol Cell. 2022. PMID: 35767320 Free PMC article.
-
Between Inflammation and Autophagy: The Role of Leptin-Adiponectin Axis in Cardiac Remodeling.J Inflamm Res. 2021 Oct 16;14:5349-5365. doi: 10.2147/JIR.S322231. eCollection 2021. J Inflamm Res. 2021. PMID: 34703273 Free PMC article. Review.
-
Mechanical strain induced phospho-proteomic signaling in uterine smooth muscle cells.J Biomech. 2018 May 17;73:99-107. doi: 10.1016/j.jbiomech.2018.03.040. Epub 2018 Mar 30. J Biomech. 2018. PMID: 29661501 Free PMC article.
-
Mechanical activation and expression of HSP27 in epithelial ovarian cancer.Sci Rep. 2024 Feb 3;14(1):2856. doi: 10.1038/s41598-024-52992-7. Sci Rep. 2024. PMID: 38310132 Free PMC article.
-
Functional Diversity of Mammalian Small Heat Shock Proteins: A Review.Cells. 2023 Jul 27;12(15):1947. doi: 10.3390/cells12151947. Cells. 2023. PMID: 37566026 Free PMC article. Review.
References
-
- Aikawa R, Nagai T, Kudoh S, Zou Y, Tanaka M, Tamura M, Akazawa H, Takano H, Nagai R, Komuro I. Integrins play a critical role in mechanical stress-induced p38 MAPK activation. Hypertension. 2002;39:233–238. - PubMed
-
- An SS, Fabry B, Mellema M, Bursac P, Gerthoffer WT, Kayyali US, Gaestel M, Shore SA, Fredberg JJ. Role of heat shock protein 27 in cytoskeletal remodeling of the airway smooth muscle cell. J Appl Physiol. 2004;96:1701–1713. - PubMed
-
- Ateshian GA, Humphrey JD. Continuum mixture models of biological growth and remodeling: past successes and future opportunities. Annu Rev Biomed Eng. 2012;14:97–111. - PubMed
-
- Benndorf R, Hayess K, Ryazantsev S, Wieske M, Behlke J, Lutsch G. Phosphorylation and supramolecular organization of murine small heat shock protein HSP25 abolish its actin polymerization-inhibiting activity. J Biol Chem. 1994;269:20780–20784. - PubMed
MeSH terms
Substances
Grants and funding
LinkOut - more resources
Full Text Sources
Other Literature Sources
Molecular Biology Databases
Research Materials
Miscellaneous