Membrane tension controls adhesion positioning at the leading edge of cells
- PMID: 28687667
- PMCID: PMC5584154
- DOI: 10.1083/jcb.201611117
Membrane tension controls adhesion positioning at the leading edge of cells
Abstract
Cell migration is dependent on adhesion dynamics and actin cytoskeleton remodeling at the leading edge. These events may be physically constrained by the plasma membrane. Here, we show that the mechanical signal produced by an increase in plasma membrane tension triggers the positioning of new rows of adhesions at the leading edge. During protrusion, as membrane tension increases, velocity slows, and the lamellipodium buckles upward in a myosin II-independent manner. The buckling occurs between the front of the lamellipodium, where nascent adhesions are positioned in rows, and the base of the lamellipodium, where a vinculin-dependent clutch couples actin to previously positioned adhesions. As membrane tension decreases, protrusion resumes and buckling disappears, until the next cycle. We propose that the mechanical signal of membrane tension exerts upstream control in mechanotransduction by periodically compressing and relaxing the lamellipodium, leading to the positioning of adhesions at the leading edge of cells.
© 2017 Pontes et al.
Figures
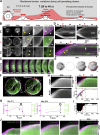
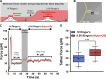
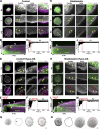
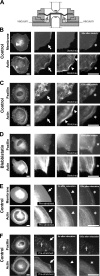
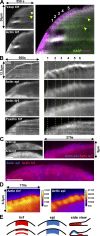
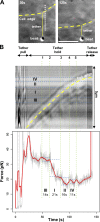
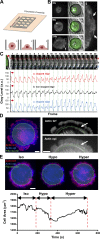
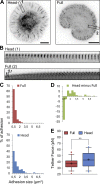
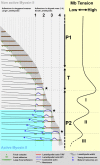
Similar articles
-
A role for actin arcs in the leading-edge advance of migrating cells.Nat Cell Biol. 2011 Apr;13(4):371-81. doi: 10.1038/ncb2205. Epub 2011 Mar 20. Nat Cell Biol. 2011. PMID: 21423177 Free PMC article.
-
Lamellipodial actin mechanically links myosin activity with adhesion-site formation.Cell. 2007 Feb 9;128(3):561-75. doi: 10.1016/j.cell.2006.12.039. Cell. 2007. PMID: 17289574 Free PMC article.
-
Weak force stalls protrusion at the leading edge of the lamellipodium.Biophys J. 2006 Mar 1;90(5):1810-20. doi: 10.1529/biophysj.105.064600. Epub 2005 Dec 2. Biophys J. 2006. PMID: 16326894 Free PMC article.
-
On the mechanisms of cortical actin flow and its role in cytoskeletal organisation of fibroblasts.Symp Soc Exp Biol. 1993;47:35-56. Symp Soc Exp Biol. 1993. PMID: 8165576 Review.
-
The comings and goings of actin: coupling protrusion and retraction in cell motility.Curr Opin Cell Biol. 2005 Oct;17(5):517-23. doi: 10.1016/j.ceb.2005.08.004. Curr Opin Cell Biol. 2005. PMID: 16099152 Review.
Cited by
-
How sticky? How tight? How hot? Imaging probes for fluid viscosity, membrane tension and temperature measurements at the cellular level.Int J Biochem Cell Biol. 2022 Dec;153:106329. doi: 10.1016/j.biocel.2022.106329. Epub 2022 Nov 3. Int J Biochem Cell Biol. 2022. PMID: 36336304 Free PMC article. Review.
-
Biomechanical Aspects of Actin Bundle Dynamics.Front Cell Dev Biol. 2020 Jun 9;8:422. doi: 10.3389/fcell.2020.00422. eCollection 2020. Front Cell Dev Biol. 2020. PMID: 32582705 Free PMC article.
-
Stiffness Sensing by Cells.Physiol Rev. 2020 Apr 1;100(2):695-724. doi: 10.1152/physrev.00013.2019. Epub 2019 Nov 21. Physiol Rev. 2020. PMID: 31751165 Free PMC article. Review.
-
Steps in Mechanotransduction Pathways that Control Cell Morphology.Annu Rev Physiol. 2019 Feb 10;81:585-605. doi: 10.1146/annurev-physiol-021317-121245. Epub 2018 Nov 7. Annu Rev Physiol. 2019. PMID: 30403543 Free PMC article. Review.
-
Physical Plasma Membrane Perturbation Using Subcellular Optogenetics Drives Integrin-Activated Cell Migration.ACS Synth Biol. 2019 Mar 15;8(3):498-510. doi: 10.1021/acssynbio.8b00356. Epub 2019 Feb 22. ACS Synth Biol. 2019. PMID: 30764607 Free PMC article.
References
-
- Burnette D.T., Shao L., Ott C., Pasapera A.M., Fischer R.S., Baird M.A., Der Loughian C., Delanoe-Ayari H., Paszek M.J., Davidson M.W., et al. . 2014. A contractile and counterbalancing adhesion system controls the 3D shape of crawling cells. J. Cell Biol. 205:83–96. 10.1083/jcb.201311104 - DOI - PMC - PubMed
Publication types
MeSH terms
Substances
LinkOut - more resources
Full Text Sources
Other Literature Sources
Research Materials