Structural Analysis of Chemokine Receptor-Ligand Interactions
- PMID: 28165741
- PMCID: PMC5483895
- DOI: 10.1021/acs.jmedchem.6b01309
Structural Analysis of Chemokine Receptor-Ligand Interactions
Abstract
This review focuses on the construction and application of structural chemokine receptor models for the elucidation of molecular determinants of chemokine receptor modulation and the structure-based discovery and design of chemokine receptor ligands. A comparative analysis of ligand binding pockets in chemokine receptors is presented, including a detailed description of the CXCR4, CCR2, CCR5, CCR9, and US28 X-ray structures, and their implication for modeling molecular interactions of chemokine receptors with small-molecule ligands, peptide ligands, and large antibodies and chemokines. These studies demonstrate how the integration of new structural information on chemokine receptors with extensive structure-activity relationship and site-directed mutagenesis data facilitates the prediction of the structure of chemokine receptor-ligand complexes that have not been crystallized. Finally, a review of structure-based ligand discovery and design studies based on chemokine receptor crystal structures and homology models illustrates the possibilities and challenges to find novel ligands for chemokine receptors.
Conflict of interest statement
The authors declare no competing financial interest.
Figures
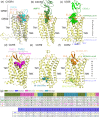
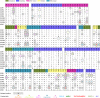
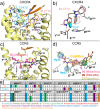
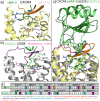
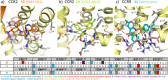
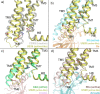
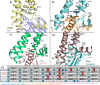
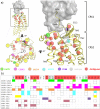
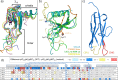
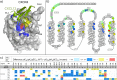
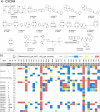
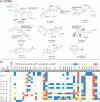
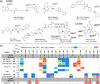
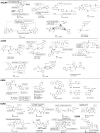
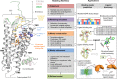
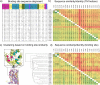
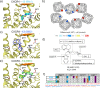
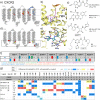
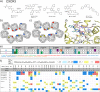
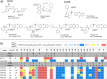
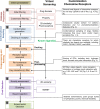
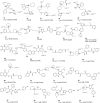
Similar articles
-
Chapter 12. The use of receptor homology modeling to facilitate the design of selective chemokine receptor antagonists.Methods Enzymol. 2009;461:249-79. doi: 10.1016/S0076-6879(09)05412-3. Methods Enzymol. 2009. PMID: 19480923
-
Closely related, yet unique: Distinct homo- and heterodimerization patterns of G protein coupled chemokine receptors and their fine-tuning by cholesterol.PLoS Comput Biol. 2018 Mar 12;14(3):e1006062. doi: 10.1371/journal.pcbi.1006062. eCollection 2018 Mar. PLoS Comput Biol. 2018. PMID: 29529028 Free PMC article.
-
Structure prediction and molecular dynamics simulations of a G-protein coupled receptor: human CCR2 receptor.J Biomol Struct Dyn. 2013;31(7):694-715. doi: 10.1080/07391102.2012.707460. Epub 2012 Aug 22. J Biomol Struct Dyn. 2013. PMID: 22909007
-
Structure-based studies of chemokine receptors.Curr Opin Struct Biol. 2013 Aug;23(4):539-46. doi: 10.1016/j.sbi.2013.05.003. Epub 2013 May 22. Curr Opin Struct Biol. 2013. PMID: 23706951 Review.
-
Chemokine receptors and HIV-1: the fusion of two major research fields.Immunol Today. 1999 Feb;20(2):89-94. doi: 10.1016/s0167-5699(98)01396-6. Immunol Today. 1999. PMID: 10098328 Review.
Cited by
-
D-Peptide-Based Probe for CXCR4-Targeted Molecular Imaging and Radionuclide Therapy.Pharmaceutics. 2021 Oct 5;13(10):1619. doi: 10.3390/pharmaceutics13101619. Pharmaceutics. 2021. PMID: 34683912 Free PMC article.
-
Heterodimers Are an Integral Component of Chemokine Signaling Repertoire.Int J Mol Sci. 2023 Jul 19;24(14):11639. doi: 10.3390/ijms241411639. Int J Mol Sci. 2023. PMID: 37511398 Free PMC article. Review.
-
CXCL12 and Its Isoforms: Different Roles in Pancreatic Cancer?J Oncol. 2019 Jun 2;2019:9681698. doi: 10.1155/2019/9681698. eCollection 2019. J Oncol. 2019. PMID: 31275385 Free PMC article. Review.
-
Role of cytokines and inflammation in heart function during health and disease.Heart Fail Rev. 2018 Sep;23(5):733-758. doi: 10.1007/s10741-018-9716-x. Heart Fail Rev. 2018. PMID: 29862462 Review.
-
Disparate Regions of the Human Chemokine CXCL10 Exhibit Broad-Spectrum Antimicrobial Activity against Biodefense and Antibiotic-Resistant Bacterial Pathogens.ACS Infect Dis. 2023 Jan 13;9(1):122-139. doi: 10.1021/acsinfecdis.2c00456. Epub 2022 Dec 7. ACS Infect Dis. 2023. PMID: 36475632 Free PMC article.
References
-
- Bongers G.; Maussang D.; Muniz L. R.; Noriega V. M.; Fraile-Ramos A.; Barker N.; Marchesi F.; Thirunarayanan N.; Vischer H. F.; Qin L.; Mayer L.; Harpaz N.; Leurs R.; Furtado G. C.; Clevers H.; Tortorella D.; Smit M. J.; Lira S. A. The cytomegalovirus-encoded chemokine receptor US28 promotes intestinal neoplasia in transgenic mice. J. Clin. Invest. 2010, 120, 3969–78. 10.1172/JCI42563. - DOI - PMC - PubMed
Publication types
MeSH terms
Substances
LinkOut - more resources
Full Text Sources
Other Literature Sources
Chemical Information