Synaptic vesicle pool-specific modification of neurotransmitter release by intravesicular free radical generation
- PMID: 27723113
- PMCID: PMC5309351
- DOI: 10.1113/JP273115
Synaptic vesicle pool-specific modification of neurotransmitter release by intravesicular free radical generation
Abstract
Key points: Synaptic transmission is mediated by the release of neurotransmitters from synaptic vesicles in response to stimulation or through the spontaneous fusion of a synaptic vesicle with the presynaptic plasma membrane. There is growing evidence that synaptic vesicles undergoing spontaneous fusion versus those fusing in response to stimuli are functionally distinct. In this study, we acutely probe the effects of intravesicular free radical generation on synaptic vesicles that fuse spontaneously or in response to stimuli. By targeting vesicles that preferentially release spontaneously, we can dissociate the effects of intravesicular free radical generation on spontaneous neurotransmission from evoked neurotransmission and vice versa. Taken together, these results further advance our knowledge of the synapse and the nature of the different synaptic vesicle pools mediating neurotransmission.
Abstract: Earlier studies suggest that spontaneous and evoked neurotransmitter release processes are maintained by synaptic vesicles which are segregated into functionally distinct pools. However, direct interrogation of the link between this putative synaptic vesicle pool heterogeneity and neurotransmission has been difficult. To examine this link, we tagged vesicles with horseradish peroxidase (HRP) - a haem-containing plant enzyme - or antibodies against synaptotagmin-1 (syt1). Filling recycling vesicles in hippocampal neurons with HRP and subsequent treatment with hydrogen peroxide (H2 O2 ) modified the properties of neurotransmitter release depending on the route of HRP uptake. While strong depolarization-induced uptake of HRP suppressed evoked release and augmented spontaneous release, HRP uptake during mild activity selectively impaired evoked release, whereas HRP uptake at rest solely potentiated spontaneous release. Expression of a luminal HRP-tagged syt1 construct and subsequent H2 O2 application resulted in a similar increase in spontaneous release and suppression as well as desynchronization of evoked release, recapitulating the canonical syt1 loss-of-function phenotype. An antibody targeting the luminal domain of syt1, on the other hand, showed that augmentation of spontaneous release and suppression of evoked release phenotypes are dissociable depending on whether the antibody uptake occurred at rest or during depolarization. Taken together, these findings indicate that vesicles that maintain spontaneous and evoked neurotransmitter release preserve their identity during recycling and syt1 function in suppression of spontaneous neurotransmission can be acutely dissociated from syt1 function to synchronize synaptic vesicle exocytosis upon stimulation.
Keywords: spontaneous neurotransmitter release; synaptic vesicle recycling; synaptotagmin.
© 2016 The Authors. The Journal of Physiology © 2016 The Physiological Society.
Figures
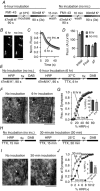
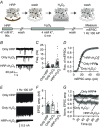
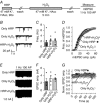
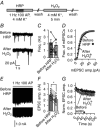
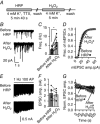
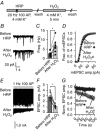
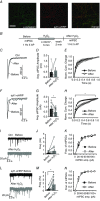
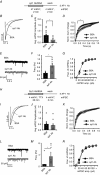
Comment in
-
A (free) radical approach reveals the physiological function of different synaptic vesicle pools.J Physiol. 2017 Feb 15;595(4):1005-1006. doi: 10.1113/JP273596. J Physiol. 2017. PMID: 28198012 Free PMC article. No abstract available.
Similar articles
-
Complexin Mutants Reveal Partial Segregation between Recycling Pathways That Drive Evoked and Spontaneous Neurotransmission.J Neurosci. 2017 Jan 11;37(2):383-396. doi: 10.1523/JNEUROSCI.1854-16.2016. J Neurosci. 2017. PMID: 28077717 Free PMC article.
-
VAMP4 Maintains a Ca2+-Sensitive Pool of Spontaneously Recycling Synaptic Vesicles.J Neurosci. 2020 Jul 8;40(28):5389-5401. doi: 10.1523/JNEUROSCI.2386-19.2020. Epub 2020 Jun 12. J Neurosci. 2020. PMID: 32532887 Free PMC article.
-
An isolated pool of vesicles recycles at rest and drives spontaneous neurotransmission.Neuron. 2005 Feb 17;45(4):563-73. doi: 10.1016/j.neuron.2004.12.056. Neuron. 2005. PMID: 15721242
-
Molecular underpinnings of synaptic vesicle pool heterogeneity.Traffic. 2015 Apr;16(4):338-64. doi: 10.1111/tra.12262. Traffic. 2015. PMID: 25620674 Free PMC article. Review.
-
TRP Channel Trafficking.In: Liedtke WB, Heller S, editors. TRP Ion Channel Function in Sensory Transduction and Cellular Signaling Cascades. Boca Raton (FL): CRC Press/Taylor & Francis; 2007. Chapter 23. In: Liedtke WB, Heller S, editors. TRP Ion Channel Function in Sensory Transduction and Cellular Signaling Cascades. Boca Raton (FL): CRC Press/Taylor & Francis; 2007. Chapter 23. PMID: 21204515 Free Books & Documents. Review.
Cited by
-
Presynaptic origins of distinct modes of neurotransmitter release.Curr Opin Neurobiol. 2018 Aug;51:119-126. doi: 10.1016/j.conb.2018.03.005. Epub 2018 Mar 26. Curr Opin Neurobiol. 2018. PMID: 29597140 Free PMC article. Review.
-
Modulation of the inwardly rectifying potassium channel Kir4.1 by the pro-invasive miR-5096 in glioblastoma cells.Oncotarget. 2017 Jun 6;8(23):37681-37693. doi: 10.18632/oncotarget.16949. Oncotarget. 2017. PMID: 28445150 Free PMC article.
-
A (free) radical approach reveals the physiological function of different synaptic vesicle pools.J Physiol. 2017 Feb 15;595(4):1005-1006. doi: 10.1113/JP273596. J Physiol. 2017. PMID: 28198012 Free PMC article. No abstract available.
-
SNT-1 Functions as the Ca2+ Sensor for Tonic and Evoked Neurotransmitter Release in Caenorhabditis Elegans.J Neurosci. 2018 Jun 6;38(23):5313-5324. doi: 10.1523/JNEUROSCI.3097-17.2018. Epub 2018 May 14. J Neurosci. 2018. PMID: 29760174 Free PMC article.
-
GFP nanobodies reveal recently-exocytosed pHluorin molecules.Sci Rep. 2019 May 23;9(1):7773. doi: 10.1038/s41598-019-44262-8. Sci Rep. 2019. PMID: 31123313 Free PMC article.
References
Publication types
MeSH terms
Substances
Grants and funding
LinkOut - more resources
Full Text Sources
Other Literature Sources