Reprogramming glucose metabolism in cancer: can it be exploited for cancer therapy?
- PMID: 27634447
- PMCID: PMC5516800
- DOI: 10.1038/nrc.2016.77
Reprogramming glucose metabolism in cancer: can it be exploited for cancer therapy?
Abstract
In recent years there has been a growing interest among cancer biologists in cancer metabolism. This Review summarizes past and recent advances in our understanding of the reprogramming of glucose metabolism in cancer cells, which is mediated by oncogenic drivers and by the undifferentiated character of cancer cells. The reprogrammed glucose metabolism in cancer cells is required to fulfil anabolic demands. This Review discusses the possibility of exploiting the reprogrammed glucose metabolism for therapeutic approaches that selectively target cancer cells.
Conflict of interest statement
The author declares no competing interests.
Figures
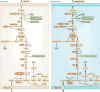
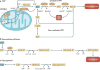
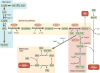
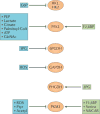
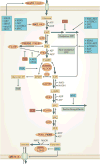
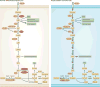
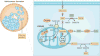
Similar articles
-
The roles of glucose metabolic reprogramming in chemo- and radio-resistance.J Exp Clin Cancer Res. 2019 May 23;38(1):218. doi: 10.1186/s13046-019-1214-z. J Exp Clin Cancer Res. 2019. PMID: 31122265 Free PMC article. Review.
-
Targeting glucose metabolism: an emerging concept for anticancer therapy.Am J Clin Oncol. 2011 Dec;34(6):628-35. doi: 10.1097/COC.0b013e3181e84dec. Am J Clin Oncol. 2011. PMID: 20805739 Review.
-
Fundamentals of cancer metabolism.Sci Adv. 2016 May 27;2(5):e1600200. doi: 10.1126/sciadv.1600200. eCollection 2016 May. Sci Adv. 2016. PMID: 27386546 Free PMC article. Review.
-
Therapeutic targeting of Myc-reprogrammed cancer cell metabolism.Cold Spring Harb Symp Quant Biol. 2011;76:369-74. doi: 10.1101/sqb.2011.76.011296. Epub 2011 Sep 29. Cold Spring Harb Symp Quant Biol. 2011. PMID: 21960526 Review.
-
Catabolic metabolism during cancer EMT.Arch Pharm Res. 2015 Mar;38(3):313-20. doi: 10.1007/s12272-015-0567-x. Epub 2015 Jan 30. Arch Pharm Res. 2015. PMID: 25634102 Review.
Cited by
-
TRIM family contribute to tumorigenesis, cancer development, and drug resistance.Exp Hematol Oncol. 2022 Oct 19;11(1):75. doi: 10.1186/s40164-022-00322-w. Exp Hematol Oncol. 2022. PMID: 36261847 Free PMC article. Review.
-
LncRNAs-circRNAs as Rising Epigenetic Binary Superstars in Regulating Lipid Metabolic Reprogramming of Cancers.Adv Sci (Weinh). 2024 Jan;11(1):e2303570. doi: 10.1002/advs.202303570. Epub 2023 Nov 8. Adv Sci (Weinh). 2024. PMID: 37939296 Free PMC article. Review.
-
Pancreatic cancer tumor organoids exhibit subtype-specific differences in metabolic profiles.Cancer Metab. 2024 Oct 3;12(1):28. doi: 10.1186/s40170-024-00357-z. Cancer Metab. 2024. PMID: 39363341 Free PMC article.
-
Tumor suppressor SMAR1 regulates PKM alternative splicing by HDAC6-mediated deacetylation of PTBP1.Cancer Metab. 2021 Apr 16;9(1):16. doi: 10.1186/s40170-021-00252-x. Cancer Metab. 2021. PMID: 33863392 Free PMC article.
-
How do cancer cells replenish their fuel supply?Cancer Rep (Hoboken). 2018 Jun;1(1):e1003. doi: 10.1002/cnr2.1003. Epub 2018 Apr 30. Cancer Rep (Hoboken). 2018. PMID: 32729259 Free PMC article. Review.
References
-
- Warburg O. The Metabolism of Tumours: Investigations from the Kaiser Wilhelm Institute for Biology, Berlin-Dahlen. Constable; 1930.
-
- Warburg O. On the origin of cancer cells. Science. 1956;123:309–314. - PubMed
-
- Warburg O. On respiratory impairment in cancer cells. Science. 1956;124:269–270. - PubMed
-
- Weinhouse S. On respiratory impairment in cancer cells. Science. 1956;124:267–269. - PubMed
Publication types
MeSH terms
Substances
Grants and funding
LinkOut - more resources
Full Text Sources
Other Literature Sources
Miscellaneous