Systems Level Analysis of the Yeast Osmo-Stat
- PMID: 27515486
- PMCID: PMC4981887
- DOI: 10.1038/srep30950
Systems Level Analysis of the Yeast Osmo-Stat
Abstract
Adaptation is an important property of living organisms enabling them to cope with environmental stress and maintaining homeostasis. Adaptation is mediated by signaling pathways responding to different stimuli. Those signaling pathways might communicate in order to orchestrate the cellular response to multiple simultaneous stimuli, a phenomenon called crosstalk. Here, we investigate possible mechanisms of crosstalk between the High Osmolarity Glycerol (HOG) and the Cell Wall Integrity (CWI) pathways in yeast, which mediate adaptation to hyper- and hypo-osmotic challenges, respectively. We combine ensemble modeling with experimental investigations to test in quantitative terms different hypotheses about the crosstalk of the HOG and the CWI pathways. Our analyses indicate that for the conditions studied i) the CWI pathway activation employs an adaptive mechanism with a variable volume-dependent threshold, in contrast to the HOG pathway, whose activation relies on a fixed volume-dependent threshold, ii) there is no or little direct crosstalk between the HOG and CWI pathways, and iii) its mainly the HOG alone mediating adaptation of cellular osmotic pressure for both hyper- as well as hypo-osmotic stress. Thus, by iteratively combining mathematical modeling with experimentation we achieved a better understanding of regulatory mechanisms of yeast osmo-homeostasis and formulated new hypotheses about osmo-sensing.
Figures
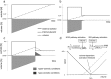
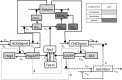
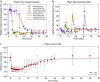
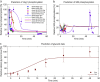
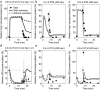
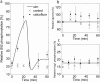
Similar articles
-
The high-osmolarity glycerol (HOG) and cell wall integrity (CWI) signalling pathways interplay: a yeast dialogue between MAPK routes.Yeast. 2010 Aug;27(8):495-502. doi: 10.1002/yea.1792. Yeast. 2010. PMID: 20641030 Review.
-
Coordination of the Cell Wall Integrity and High-Osmolarity Glycerol Pathways in Response to Ethanol Stress in Saccharomyces cerevisiae.Appl Environ Microbiol. 2019 Jul 18;85(15):e00551-19. doi: 10.1128/AEM.00551-19. Print 2019 Aug 1. Appl Environ Microbiol. 2019. PMID: 31101611 Free PMC article.
-
Participation of CWI, HOG and Calcineurin pathways in the tolerance of Saccharomyces cerevisiae to low pH by inorganic acid.J Appl Microbiol. 2012 Sep;113(3):629-40. doi: 10.1111/j.1365-2672.2012.05362.x. Epub 2012 Jul 17. J Appl Microbiol. 2012. PMID: 22702539
-
Response to high osmotic conditions and elevated temperature in Saccharomyces cerevisiae is controlled by intracellular glycerol and involves coordinate activity of MAP kinase pathways.Microbiology (Reading). 2003 May;149(Pt 5):1193-1204. doi: 10.1099/mic.0.26110-0. Microbiology (Reading). 2003. PMID: 12724381
-
Osmoregulation in Saccharomyces cerevisiae via mechanisms other than the high-osmolarity glycerol pathway.Microbiology (Reading). 2016 Sep;162(9):1511-1526. doi: 10.1099/mic.0.000360. Epub 2016 Aug 23. Microbiology (Reading). 2016. PMID: 27557593 Review.
Cited by
-
Yeast two- and three-species hybrids and high-sugar fermentation.Microb Biotechnol. 2019 Nov;12(6):1101-1108. doi: 10.1111/1751-7915.13390. Epub 2019 Mar 5. Microb Biotechnol. 2019. PMID: 30838806 Free PMC article. Review.
-
Draft Genome Sequence of the Yeast Saccharomyces cerevisiae GUJ105 From Gujarat, India.Genome Announc. 2016 Dec 1;4(6):e01315-16. doi: 10.1128/genomeA.01315-16. Genome Announc. 2016. PMID: 27908989 Free PMC article.
-
Exploring the role of stromal osmoregulation in cancer and disease using executable modelling.Nat Commun. 2018 Aug 1;9(1):3011. doi: 10.1038/s41467-018-05414-y. Nat Commun. 2018. PMID: 30069015 Free PMC article.
-
Heat-stress triggers MAPK crosstalk to turn on the hyperosmotic response pathway.Sci Rep. 2018 Oct 11;8(1):15168. doi: 10.1038/s41598-018-33203-6. Sci Rep. 2018. PMID: 30310096 Free PMC article.
-
Investigation of osmotic shock effect on pulsed electric field treated S. cerevisiae yeast cells.Sci Rep. 2023 Jun 29;13(1):10573. doi: 10.1038/s41598-023-37719-4. Sci Rep. 2023. PMID: 37386124 Free PMC article.
References
Publication types
MeSH terms
Substances
LinkOut - more resources
Full Text Sources
Other Literature Sources
Molecular Biology Databases