Three-dimensional-printed gas dynamic virtual nozzles for x-ray laser sample delivery
- PMID: 27410079
- PMCID: PMC5025224
- DOI: 10.1364/OE.24.011515
Three-dimensional-printed gas dynamic virtual nozzles for x-ray laser sample delivery
Abstract
Reliable sample delivery is essential to biological imaging using X-ray Free Electron Lasers (XFELs). Continuous injection using the Gas Dynamic Virtual Nozzle (GDVN) has proven valuable, particularly for time-resolved studies. However, many important aspects of GDVN functionality have yet to be thoroughly understood and/or refined due to fabrication limitations. We report the application of 2-photon polymerization as a form of high-resolution 3D printing to fabricate high-fidelity GDVNs with submicron resolution. This technique allows rapid prototyping of a wide range of different types of nozzles from standard CAD drawings and optimization of crucial dimensions for optimal performance. Three nozzles were tested with pure water to determine general nozzle performance and reproducibility, with nearly reproducible off-axis jetting being the result. X-ray tomography and index matching were successfully used to evaluate the interior nozzle structures and identify the cause of off-axis jetting. Subsequent refinements to fabrication resulted in straight jetting. A performance test of printed nozzles at an XFEL provided high quality femtosecond diffraction patterns.
Figures
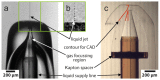
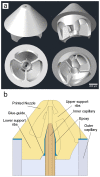
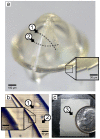
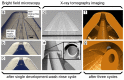
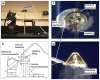
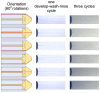
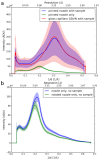
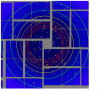
Similar articles
-
Electrically stimulated droplet injector for reduced sample consumption in serial crystallography.Biophys Rep (N Y). 2022 Sep 29;2(4):100081. doi: 10.1016/j.bpr.2022.100081. eCollection 2022 Dec 14. Biophys Rep (N Y). 2022. PMID: 36425668 Free PMC article.
-
3D printing of gas-dynamic virtual nozzles and optical characterization of high-speed microjets.Opt Express. 2020 Jul 20;28(15):21749-21765. doi: 10.1364/OE.390131. Opt Express. 2020. PMID: 32752448 Free PMC article.
-
3D printed devices and infrastructure for liquid sample delivery at the European XFEL.J Synchrotron Radiat. 2022 Mar 1;29(Pt 2):331-346. doi: 10.1107/S1600577521013370. Epub 2022 Feb 15. J Synchrotron Radiat. 2022. PMID: 35254295 Free PMC article.
-
Recent Advances and Future Perspectives on Microfluidic Mix-and-Jet Sample Delivery Devices.Micromachines (Basel). 2021 May 7;12(5):531. doi: 10.3390/mi12050531. Micromachines (Basel). 2021. PMID: 34067131 Free PMC article. Review.
-
A Bright Future for Serial Femtosecond Crystallography with XFELs.Trends Biochem Sci. 2017 Sep;42(9):749-762. doi: 10.1016/j.tibs.2017.06.007. Epub 2017 Jul 18. Trends Biochem Sci. 2017. PMID: 28733116 Free PMC article. Review.
Cited by
-
Electrically stimulated droplet injector for reduced sample consumption in serial crystallography.Biophys Rep (N Y). 2022 Sep 29;2(4):100081. doi: 10.1016/j.bpr.2022.100081. eCollection 2022 Dec 14. Biophys Rep (N Y). 2022. PMID: 36425668 Free PMC article.
-
Development of Container Free Sample Exposure for Synchrotron X-ray Footprinting.Anal Chem. 2020 Jan 7;92(1):1565-1573. doi: 10.1021/acs.analchem.9b04849. Epub 2019 Dec 16. Anal Chem. 2020. PMID: 31790200 Free PMC article.
-
Ultracompact 3D microfluidics for time-resolved structural biology.Nat Commun. 2020 Jan 31;11(1):657. doi: 10.1038/s41467-020-14434-6. Nat Commun. 2020. PMID: 32005876 Free PMC article.
-
Strategies for sample delivery for femtosecond crystallography.Acta Crystallogr D Struct Biol. 2019 Feb 1;75(Pt 2):160-177. doi: 10.1107/S2059798318017953. Epub 2019 Feb 19. Acta Crystallogr D Struct Biol. 2019. PMID: 30821705 Free PMC article. Review.
-
3D-glass molds for facile production of complex droplet microfluidic chips.Biomicrofluidics. 2018 Apr 3;12(2):024115. doi: 10.1063/1.5013325. eCollection 2018 Mar. Biomicrofluidics. 2018. PMID: 29657658 Free PMC article.
References
-
- McNeil B. W. J., Thompson N. R., “X-ray free-electron lasers,” Nat. Photonics 4(12), 814–821 (2010).10.1038/nphoton.2010.239 - DOI
-
- Chapman H. N., Fromme P., Barty A., White T. A., Kirian R. A., Aquila A., Hunter M. S., Schulz J., DePonte D. P., Weierstall U., Doak R. B., Maia F. R. N. C., Martin A. V., Schlichting I., Lomb L., Coppola N., Shoeman R. L., Epp S. W., Hartmann R., Rolles D., Rudenko A., Foucar L., Kimmel N., Weidenspointner G., Holl P., Liang M., Barthelmess M., Caleman C., Boutet S., Bogan M. J., Krzywinski J., Bostedt C., Bajt S., Gumprecht L., Rudek B., Erk B., Schmidt C., Hömke A., Reich C., Pietschner D., Strüder L., Hauser G., Gorke H., Ullrich J., Herrmann S., Schaller G., Schopper F., Soltau H., Kühnel K. U., Messerschmidt M., Bozek J. D., Hau-Riege S. P., Frank M., Hampton C. Y., Sierra R. G., Starodub D., Williams G. J., Hajdu J., Timneanu N., Seibert M. M., Andreasson J., Rocker A., Jönsson O., Svenda M., Stern S., Nass K., Andritschke R., Schröter C. D., Krasniqi F., Bott M., Schmidt K. E., Wang X., Grotjohann I., Holton J. M., Barends T. R. M., Neutze R., Marchesini S., Fromme R., Schorb S., Rupp D., Adolph M., Gorkhover T., Andersson I., Hirsemann H., Potdevin G., Graafsma H., Nilsson B., Spence J. C. H., “Femtosecond X-ray protein nanocrystallography,” Nature 470(7332), 73–77 (2011).10.1038/nature09750 - DOI - PMC - PubMed
-
- Tenboer J., Basu S., Zatsepin N., Pande K., Milathianaki D., Frank M., Hunter M., Boutet S., Williams G. J., Koglin J. E., Oberthuer D., Heymann M., Kupitz C., Conrad C., Coe J., Roy-Chowdhury S., Weierstall U., James D., Wang D., Grant T., Barty A., Yefanov O., Scales J., Gati C., Seuring C., Srajer V., Henning R., Schwander P., Fromme R., Ourmazd A., Moffat K., Van Thor J. J., Spence J. C. H., Fromme P., Chapman H. N., Schmidt M., “Time-resolved serial crystallography captures high-resolution intermediates of photoactive yellow protein,” Science 346(6214), 1242–1246 (2014).10.1126/science.1259357 - DOI - PMC - PubMed
-
- Gañán-Calvo A. M., “Generation of steady liquid microthreads and micron-sized monodisperse sprays in gas streams,” Phys. Rev. Lett. 80(2), 285–288 (1998).10.1103/PhysRevLett.80.285 - DOI
Grants and funding
LinkOut - more resources
Full Text Sources
Other Literature Sources
Miscellaneous