λ Recombination and Recombineering
- PMID: 27223821
- PMCID: PMC11575712
- DOI: 10.1128/ecosalplus.ESP-0011-2015
λ Recombination and Recombineering
Abstract
The bacteriophage λ Red homologous recombination system has been studied over the past 50 years as a model system to define the mechanistic details of how organisms exchange DNA segments that share extended regions of homology. The λ Red system proved useful as a system to study because recombinants could be easily generated by co-infection of genetically marked phages. What emerged from these studies was the recognition that replication of phage DNA was required for substantial Red-promoted recombination in vivo, and the critical role that double-stranded DNA ends play in allowing the Red proteins access to the phage DNA chromosomes. In the past 16 years, however, the λ Red recombination system has gained a new notoriety. When expressed independently of other λ functions, the Red system is able to promote recombination of linear DNA containing limited regions of homology (∼50 bp) with the Escherichia coli chromosome, a process known as recombineering. This review explains how the Red system works during a phage infection, and how it is utilized to make chromosomal modifications of E. coli with such efficiency that it changed the nature and number of genetic manipulations possible, leading to advances in bacterial genomics, metabolic engineering, and eukaryotic genetics.
Figures
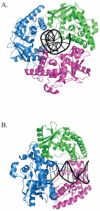
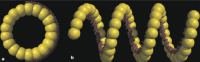
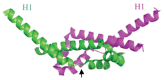
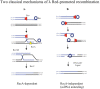
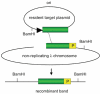
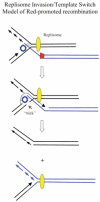
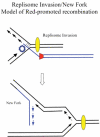
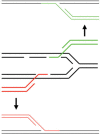
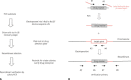
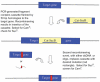
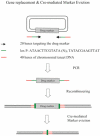
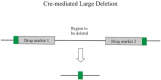
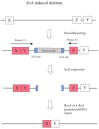
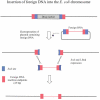
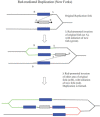
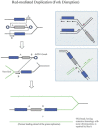
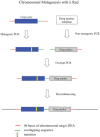
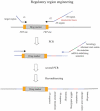
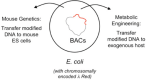
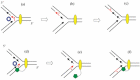
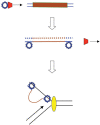
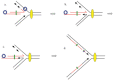
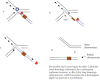
Similar articles
-
Examining a DNA Replication Requirement for Bacteriophage λ Red- and Rac Prophage RecET-Promoted Recombination in Escherichia coli.mBio. 2016 Sep 13;7(5):e01443-16. doi: 10.1128/mBio.01443-16. mBio. 2016. PMID: 27624131 Free PMC article.
-
Multicopy plasmid modification with phage lambda Red recombineering.Plasmid. 2007 Sep;58(2):148-58. doi: 10.1016/j.plasmid.2007.03.001. Epub 2007 Apr 16. Plasmid. 2007. PMID: 17434584 Free PMC article.
-
Modifying bacteriophage lambda with recombineering.Methods Mol Biol. 2009;501:239-51. doi: 10.1007/978-1-60327-164-6_21. Methods Mol Biol. 2009. PMID: 19066825
-
Genetic engineering using homologous recombination.Annu Rev Genet. 2002;36:361-88. doi: 10.1146/annurev.genet.36.061102.093104. Epub 2002 Jun 11. Annu Rev Genet. 2002. PMID: 12429697 Review.
-
[Recombineering and its application].Yi Chuan Xue Bao. 2003 Oct;30(10):983-8. Yi Chuan Xue Bao. 2003. PMID: 14669518 Review. Chinese.
Cited by
-
High-throughput functional variant screens via in vivo production of single-stranded DNA.Proc Natl Acad Sci U S A. 2021 May 4;118(18):e2018181118. doi: 10.1073/pnas.2018181118. Proc Natl Acad Sci U S A. 2021. PMID: 33906944 Free PMC article.
-
Optimization of a Lambda-RED Recombination Method for Rapid Gene Deletion in Human Cytomegalovirus.Int J Mol Sci. 2021 Sep 29;22(19):10558. doi: 10.3390/ijms221910558. Int J Mol Sci. 2021. PMID: 34638896 Free PMC article.
-
A Robust One-Step Recombineering System for Enterohemorrhagic Escherichia coli.Microorganisms. 2022 Aug 23;10(9):1689. doi: 10.3390/microorganisms10091689. Microorganisms. 2022. PMID: 36144292 Free PMC article.
-
Assessing the Role of Bacterial Innate and Adaptive Immunity as Barriers to Conjugative Plasmids.Mol Biol Evol. 2024 Oct 4;41(10):msae207. doi: 10.1093/molbev/msae207. Mol Biol Evol. 2024. PMID: 39382385 Free PMC article.
-
CRISPR/Cas9 recombineering-mediated deep mutational scanning of essential genes in Escherichia coli.Mol Syst Biol. 2020 Mar;16(3):e9265. doi: 10.15252/msb.20199265. Mol Syst Biol. 2020. PMID: 32175691 Free PMC article.
References
-
- Jacob F, Wollman E. 1954. Etude genetique d’un bacteriophage tempere d’Echerichia coli. I. Le systeme genetique du bacteriophage lambda. Ann Inst Pasteur 87:653–674. - PubMed
Publication types
MeSH terms
Substances
LinkOut - more resources
Full Text Sources
Other Literature Sources
Miscellaneous