Molecular Interactions between Tarantula Toxins and Low-Voltage-Activated Calcium Channels
- PMID: 27045173
- PMCID: PMC4820701
- DOI: 10.1038/srep23894
Molecular Interactions between Tarantula Toxins and Low-Voltage-Activated Calcium Channels
Abstract
Few gating-modifier toxins have been reported to target low-voltage-activated (LVA) calcium channels, and the structural basis of toxin sensitivity remains incompletely understood. Studies of voltage-gated potassium (Kv) channels have identified the S3b-S4 "paddle motif," which moves at the protein-lipid interface to drive channel opening, as the target for these amphipathic neurotoxins. Voltage-gated calcium (Cav) channels contain four homologous voltage sensor domains, suggesting multiple toxin binding sites. We show here that the S3-S4 segments within Cav3.1 can be transplanted into Kv2.1 to examine their individual contributions to voltage sensing and pharmacology. With these results, we now have a more complete picture of the conserved nature of the paddle motif in all three major voltage-gated ion channel types (Kv, Nav, and Cav). When screened with tarantula toxins, the four paddle sequences display distinct toxin binding properties, demonstrating that gating-modifier toxins can bind to Cav channels in a domain specific fashion. Domain III was the most commonly and strongly targeted, and mutagenesis revealed an acidic residue that is important for toxin binding. We also measured the lipid partitioning strength of all toxins tested and observed a positive correlation with their inhibition of Cav3.1, suggesting a key role for membrane partitioning.
Figures
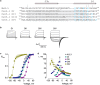
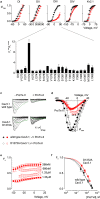
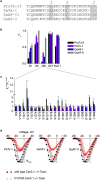
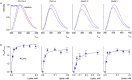
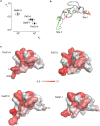
Similar articles
-
Analysis of the interaction of tarantula toxin Jingzhaotoxin-III (β-TRTX-Cj1α) with the voltage sensor of Kv2.1 uncovers the molecular basis for cross-activities on Kv2.1 and Nav1.5 channels.Biochemistry. 2013 Oct 22;52(42):7439-48. doi: 10.1021/bi4006418. Epub 2013 Oct 7. Biochemistry. 2013. PMID: 24044413
-
Interactions between lipids and voltage sensor paddles detected with tarantula toxins.Nat Struct Mol Biol. 2009 Oct;16(10):1080-5. doi: 10.1038/nsmb.1679. Epub 2009 Sep 27. Nat Struct Mol Biol. 2009. PMID: 19783984 Free PMC article.
-
Tarantula toxins use common surfaces for interacting with Kv and ASIC ion channels.Elife. 2015 May 7;4:e06774. doi: 10.7554/eLife.06774. Elife. 2015. PMID: 25948544 Free PMC article.
-
Voltage-gated ion channels and gating modifier toxins.Toxicon. 2007 Feb;49(2):124-41. doi: 10.1016/j.toxicon.2006.09.022. Epub 2006 Sep 28. Toxicon. 2007. PMID: 17239913 Review.
-
The interaction of spider gating modifier peptides with voltage-gated potassium channels.Toxicon. 2007 Feb;49(2):285-92. doi: 10.1016/j.toxicon.2006.09.015. Epub 2006 Sep 27. Toxicon. 2007. PMID: 17113615 Review.
Cited by
-
The Drosophila Gr28bD product is a non-specific cation channel that can be used as a novel thermogenetic tool.Sci Rep. 2018 Jan 17;8(1):901. doi: 10.1038/s41598-017-19065-4. Sci Rep. 2018. PMID: 29343813 Free PMC article.
-
µ-Theraphotoxin Pn3a inhibition of CaV3.3 channels reveals a novel isoform-selective drug binding site.Elife. 2022 Jul 20;11:e74040. doi: 10.7554/eLife.74040. Elife. 2022. PMID: 35858123 Free PMC article.
-
Using voltage-sensor toxins and their molecular targets to investigate NaV 1.8 gating.J Physiol. 2018 May 15;596(10):1863-1872. doi: 10.1113/JP275102. Epub 2018 Jan 6. J Physiol. 2018. PMID: 29193176 Free PMC article. Review.
-
Insect-Active Toxins with Promiscuous Pharmacology from the African Theraphosid Spider Monocentropus balfouri.Toxins (Basel). 2017 May 5;9(5):155. doi: 10.3390/toxins9050155. Toxins (Basel). 2017. PMID: 28475112 Free PMC article.
-
Multitarget nociceptor sensitization by a promiscuous peptide from the venom of the King Baboon spider.Proc Natl Acad Sci U S A. 2022 Feb 1;119(5):e2110932119. doi: 10.1073/pnas.2110932119. Proc Natl Acad Sci U S A. 2022. PMID: 35074873 Free PMC article.
References
Publication types
MeSH terms
Substances
Grants and funding
LinkOut - more resources
Full Text Sources
Other Literature Sources