Biological Nanomotors with a Revolution, Linear, or Rotation Motion Mechanism
- PMID: 26819321
- PMCID: PMC4771369
- DOI: 10.1128/MMBR.00056-15
Biological Nanomotors with a Revolution, Linear, or Rotation Motion Mechanism
Abstract
The ubiquitous biological nanomotors were classified into two categories in the past: linear and rotation motors. In 2013, a third type of biomotor, revolution without rotation (http://rnanano.osu.edu/movie.html), was discovered and found to be widespread among bacteria, eukaryotic viruses, and double-stranded DNA (dsDNA) bacteriophages. This review focuses on recent findings about various aspects of motors, including chirality, stoichiometry, channel size, entropy, conformational change, and energy usage rate, in a variety of well-studied motors, including FoF1 ATPase, helicases, viral dsDNA-packaging motors, bacterial chromosome translocases, myosin, kinesin, and dynein. In particular, dsDNA translocases are used to illustrate how these features relate to the motion mechanism and how nature elegantly evolved a revolution mechanism to avoid coiling and tangling during lengthy dsDNA genome transportation in cell division. Motor chirality and channel size are two factors that distinguish rotation motors from revolution motors. Rotation motors use right-handed channels to drive the right-handed dsDNA, similar to the way a nut drives the bolt with threads in same orientation; revolution motors use left-handed motor channels to revolve the right-handed dsDNA. Rotation motors use small channels (<2 nm in diameter) for the close contact of the channel wall with single-stranded DNA (ssDNA) or the 2-nm dsDNA bolt; revolution motors use larger channels (>3 nm) with room for the bolt to revolve. Binding and hydrolysis of ATP are linked to different conformational entropy changes in the motor that lead to altered affinity for the substrate and allow work to be done, for example, helicase unwinding of DNA or translocase directional movement of DNA.
Copyright © 2016, American Society for Microbiology. All Rights Reserved.
Figures
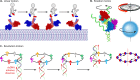
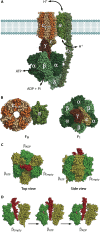
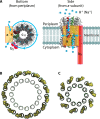
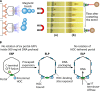
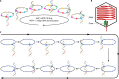
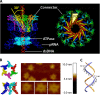
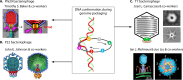
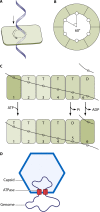
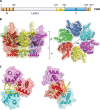
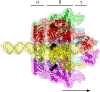
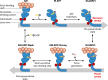
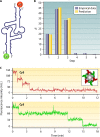
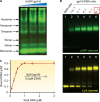
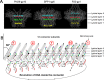
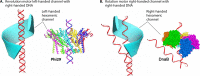
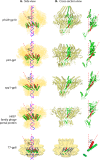
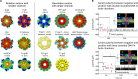
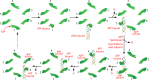
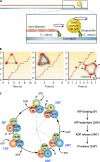
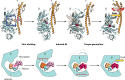
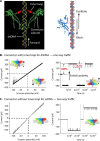
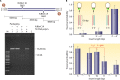
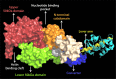
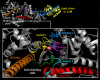
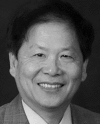
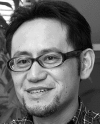
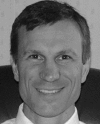
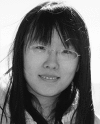
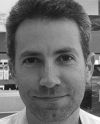
Similar articles
-
Common mechanisms of DNA translocation motors in bacteria and viruses using one-way revolution mechanism without rotation.Biotechnol Adv. 2014 Jul-Aug;32(4):853-72. doi: 10.1016/j.biotechadv.2014.01.006. Biotechnol Adv. 2014. PMID: 24913057 Free PMC article. Review.
-
Finding of widespread viral and bacterial revolution dsDNA translocation motors distinct from rotation motors by channel chirality and size.Cell Biosci. 2014 Jun 1;4:30. doi: 10.1186/2045-3701-4-30. eCollection 2014. Cell Biosci. 2014. PMID: 24940480 Free PMC article.
-
Development of Potent Antiviral Drugs Inspired by Viral Hexameric DNA-Packaging Motors with Revolving Mechanism.J Virol. 2016 Aug 26;90(18):8036-46. doi: 10.1128/JVI.00508-16. Print 2016 Sep 15. J Virol. 2016. PMID: 27356896 Free PMC article. Review.
-
Two classes of nucleic acid translocation motors: rotation and revolution without rotation.Cell Biosci. 2014 Sep 16;4(1):54. doi: 10.1186/2045-3701-4-54. eCollection 2014. Cell Biosci. 2014. PMID: 25276341 Free PMC article.
-
Discovery of a new motion mechanism of biomotors similar to the earth revolving around the sun without rotation.Virology. 2013 Nov;446(1-2):133-43. doi: 10.1016/j.virol.2013.07.025. Epub 2013 Aug 27. Virology. 2013. PMID: 24074575 Free PMC article. Review.
Cited by
-
Simulating biological surface dynamics in high-speed atomic force microscopy experiments.Biophys Rev. 2023 Dec 23;15(6):2069-2079. doi: 10.1007/s12551-023-01169-z. eCollection 2023 Dec. Biophys Rev. 2023. PMID: 38192349 Free PMC article.
-
Large conformational changes of a highly dynamic pre-protein binding domain in SecA.Commun Biol. 2018 Sep 3;1:130. doi: 10.1038/s42003-018-0133-4. eCollection 2018. Commun Biol. 2018. PMID: 30272009 Free PMC article.
-
Optical tweezer and TIRF microscopy for single molecule manipulation of RNA/DNA nanostructures including their rubbery property and single molecule counting.Biophys Rep. 2021 Dec 31;7(6):449-474. doi: 10.52601/bpr.2021.210003. Biophys Rep. 2021. PMID: 37288367 Free PMC article.
-
F1-ATPase Rotary Mechanism: Interpreting Results of Diverse Experimental Modes With an Elastic Coupling Theory.Front Microbiol. 2022 Apr 22;13:861855. doi: 10.3389/fmicb.2022.861855. eCollection 2022. Front Microbiol. 2022. PMID: 35531282 Free PMC article. Review.
-
Quantitative Study of the Chiral Organization of the Phage Genome Induced by the Packaging Motor.Biophys J. 2020 May 5;118(9):2103-2116. doi: 10.1016/j.bpj.2020.03.030. Epub 2020 Apr 14. Biophys J. 2020. PMID: 32353255 Free PMC article.
References
-
- McCluskey R. 2013. The Sixth Scottish University. The Scots colleges abroad: 1575 to 1799. Catholic Histor Rev 99:360–361.
Publication types
MeSH terms
Substances
Grants and funding
LinkOut - more resources
Full Text Sources
Other Literature Sources
Miscellaneous