Principles of nanoparticle design for overcoming biological barriers to drug delivery
- PMID: 26348965
- PMCID: PMC4978509
- DOI: 10.1038/nbt.3330
Principles of nanoparticle design for overcoming biological barriers to drug delivery
Abstract
Biological barriers to drug transport prevent successful accumulation of nanotherapeutics specifically at diseased sites, limiting efficacious responses in disease processes ranging from cancer to inflammation. Although substantial research efforts have aimed to incorporate multiple functionalities and moieties within the overall nanoparticle design, many of these strategies fail to adequately address these barriers. Obstacles, such as nonspecific distribution and inadequate accumulation of therapeutics, remain formidable challenges to drug developers. A reimagining of conventional nanoparticles is needed to successfully negotiate these impediments to drug delivery. Site-specific delivery of therapeutics will remain a distant reality unless nanocarrier design takes into account the majority, if not all, of the biological barriers that a particle encounters upon intravenous administration. By successively addressing each of these barriers, innovative design features can be rationally incorporated that will create a new generation of nanotherapeutics, realizing a paradigmatic shift in nanoparticle-based drug delivery.
Figures
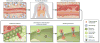
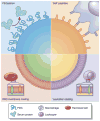
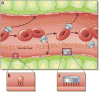
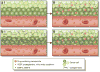
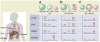
Similar articles
-
Editorial: Brave new world - Focus on nanomedicine.Biochem Biophys Res Commun. 2015 Dec 18;468(3):409-10. doi: 10.1016/j.bbrc.2015.10.135. Epub 2015 Oct 28. Biochem Biophys Res Commun. 2015. PMID: 26518649 No abstract available.
-
Nanobiotechnology and its applications in drug delivery system: a review.IET Nanobiotechnol. 2015 Dec;9(6):396-400. doi: 10.1049/iet-nbt.2014.0062. IET Nanobiotechnol. 2015. PMID: 26647817 Review.
-
Nanotechnology based targeted drug delivery.Annu Int Conf IEEE Eng Med Biol Soc. 2010;2010:3731-2. doi: 10.1109/IEMBS.2010.5627506. Annu Int Conf IEEE Eng Med Biol Soc. 2010. PMID: 21096863
-
Biophysicochemical perspective of nanoparticle compatibility: a critically ignored parameter in nanomedicine.J Nanosci Nanotechnol. 2014 Jan;14(1):402-14. doi: 10.1166/jnn.2014.8747. J Nanosci Nanotechnol. 2014. PMID: 24730271 Review.
-
Multifunctional nanocarriers for simultaneous encapsulation of hydrophobic and hydrophilic drugs in cancer treatment.Nanomedicine (Lond). 2014 Jul;9(10):1499-515. doi: 10.2217/nnm.14.97. Nanomedicine (Lond). 2014. PMID: 25253498 Review.
Cited by
-
Mapping global research landscape and trend of nano-drug delivery system for urological cancers: a bibliometric analysis.Nanomedicine (Lond). 2024;19(26):2139-2157. doi: 10.1080/17435889.2024.2391267. Epub 2024 Sep 3. Nanomedicine (Lond). 2024. PMID: 39225560 Free PMC article.
-
Nanomedicine for acute respiratory distress syndrome: The latest application, targeting strategy, and rational design.Acta Pharm Sin B. 2021 Oct;11(10):3060-3091. doi: 10.1016/j.apsb.2021.04.023. Epub 2021 May 7. Acta Pharm Sin B. 2021. PMID: 33977080 Free PMC article. Review.
-
In vivo targeting capacities of different nanoparticles to prostate tissues based on a mouse model of chronic bacterial prostatitis.Front Bioeng Biotechnol. 2022 Oct 6;10:1021385. doi: 10.3389/fbioe.2022.1021385. eCollection 2022. Front Bioeng Biotechnol. 2022. PMID: 36277385 Free PMC article.
-
A Targeted and Protease-Activated Genetically Encoded Melittin-Containing Particle for the Treatment of Cutaneous and Visceral Leishmaniasis.ACS Appl Mater Interfaces. 2024 Sep 18;16(37):49148-49163. doi: 10.1021/acsami.4c10426. Epub 2024 Sep 6. ACS Appl Mater Interfaces. 2024. PMID: 39240583 Free PMC article.
-
Nanozyme-laden intelligent macrophage EXPRESS amplifying cancer photothermal-starvation therapy by responsive stimulation.Mater Today Bio. 2022 Sep 6;16:100421. doi: 10.1016/j.mtbio.2022.100421. eCollection 2022 Dec. Mater Today Bio. 2022. PMID: 36105675 Free PMC article.
References
-
- Kola I, Landis J. Can the pharmaceutical industry reduce attrition rates? Nat Rev Drug Discov. 2004;3:711–716. - PubMed
-
- Torchilin VP. Recent advances with liposomes as pharmaceutical carriers. Nat Rev Drug Discov. 2005;4:145–160. - PubMed
-
- Matsumura Y, Maeda H. A new concept for macromolecular therapeutics in cancer chemotherapy: mechanism of tumoritropic accumulation of proteins and the antitumor agent smancs. Cancer Res. 1986;46:6387–6392. A seminal work on the EPR effect in cancer that essentially laid the foundation for the use of nanotherapeutics, including liposomes and polymer-drug conjugates, as treatment modalities. - PubMed
-
- Maeda H, Nakamura H, Fang J. The EPR effect for macromolecular drug delivery to solid tumors: Improvement of tumor uptake, lowering of systemic toxicity, and distinct tumor imaging in vivo. Adv Drug Deliv Rev. 2013;65:71–79. - PubMed
-
- Azzopardi EA, Ferguson EL, Thomas DW. The enhanced permeability retention effect: a new paradigm for drug targeting in infection. J Antimicrob Chemother. 2013;68:257–274. - PubMed
Publication types
MeSH terms
Substances
Grants and funding
LinkOut - more resources
Full Text Sources
Other Literature Sources