Photochemistry and Photobiology of the Spore Photoproduct: A 50-Year Journey
- PMID: 26265564
- PMCID: PMC4631623
- DOI: 10.1111/php.12506
Photochemistry and Photobiology of the Spore Photoproduct: A 50-Year Journey
Abstract
Fifty years ago, a new thymine dimer was discovered as the dominant DNA photolesion in UV-irradiated bacterial spores [Donnellan, J. E. & Setlow R. B. (1965) Science, 149, 308-310], which was later named the spore photoproduct (SP). Formation of SP is due to the unique environment in the spore core that features low hydration levels favoring an A-DNA conformation, high levels of calcium dipicolinate that acts as a photosensitizer, and DNA saturation with small, acid-soluble proteins that alters DNA structure and reduces side reactions. In vitro studies reveal that any of these factors alone can promote SP formation; however, SP formation is usually accompanied by the production of other DNA photolesions. Therefore, the nearly exclusive SP formation in spores is due to the combined effects of these three factors. Spore photoproduct photoreaction is proved to occur via a unique H-atom transfer mechanism between the two involved thymine residues. Successful incorporation of SP into an oligonucleotide has been achieved via organic synthesis, which enables structural studies that reveal minor conformational changes in the SP-containing DNA. Here, we review the progress on SP photochemistry and photobiology in the past 50 years, which indicates a very rich SP photobiology that may exist beyond endospores.
© 2015 The American Society of Photobiology.
Figures
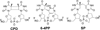
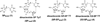
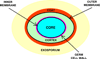
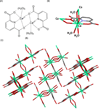
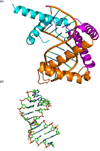
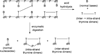
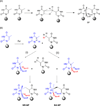
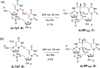
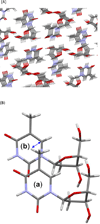
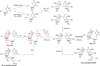
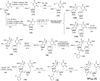
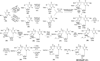
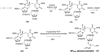
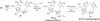
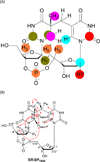
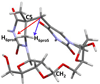
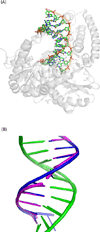
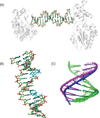
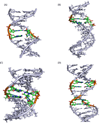
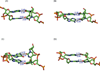
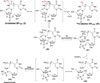
Similar articles
-
Spore photoproduct within DNA is a surprisingly poor substrate for its designated repair enzyme-The spore photoproduct lyase.DNA Repair (Amst). 2017 May;53:31-42. doi: 10.1016/j.dnarep.2016.11.005. Epub 2017 Mar 6. DNA Repair (Amst). 2017. PMID: 28320593 Free PMC article.
-
High-pressure liquid chromatography assay for quantitatively monitoring spore photoproduct repair mediated by spore photoproduct lyase during germination of uv-irradiated Bacillus subtilis spores.Anal Biochem. 1994 Aug 15;221(1):61-5. doi: 10.1006/abio.1994.1379. Anal Biochem. 1994. PMID: 7985805
-
Resistance of spores of Bacillus species to ultraviolet light.Environ Mol Mutagen. 2001;38(2-3):97-104. doi: 10.1002/em.1058. Environ Mol Mutagen. 2001. PMID: 11746741 Review.
-
Spore photoproduct (SP) lyase from Bacillus subtilis specifically binds to and cleaves SP (5-thyminyl-5,6-dihydrothymine) but not cyclobutane pyrimidine dimers in UV-irradiated DNA.J Bacteriol. 2000 Nov;182(22):6412-7. doi: 10.1128/JB.182.22.6412-6417.2000. J Bacteriol. 2000. PMID: 11053385 Free PMC article.
-
Spores of Bacillus subtilis: their resistance to and killing by radiation, heat and chemicals.J Appl Microbiol. 2006 Sep;101(3):514-25. doi: 10.1111/j.1365-2672.2005.02736.x. J Appl Microbiol. 2006. PMID: 16907802 Review.
Cited by
-
Raman spectroscopy reveals alteration of spore compositions under different nutritional conditions in Lysinibacillus boronitolerans YS11.J Microbiol. 2021 May;59(5):491-499. doi: 10.1007/s12275-021-0679-6. Epub 2021 Mar 29. J Microbiol. 2021. PMID: 33779962
-
On the Use of the Intrinsic DNA Fluorescence for Monitoring Its Damage: A Contribution from Fundamental Studies.ACS Omega. 2024 Jun 13;9(25):26826-26837. doi: 10.1021/acsomega.4c02256. eCollection 2024 Jun 25. ACS Omega. 2024. PMID: 38947837 Free PMC article. Review.
-
Nucleoid-associated proteins shape chromatin structure and transcriptional regulation across the bacterial kingdom.Transcription. 2021 Aug;12(4):182-218. doi: 10.1080/21541264.2021.1973865. Epub 2021 Sep 9. Transcription. 2021. PMID: 34499567 Free PMC article. Review.
-
Evolutionary Origins of DNA Repair Pathways: Role of Oxygen Catastrophe in the Emergence of DNA Glycosylases.Cells. 2021 Jun 24;10(7):1591. doi: 10.3390/cells10071591. Cells. 2021. PMID: 34202661 Free PMC article. Review.
-
Spore photoproduct within DNA is a surprisingly poor substrate for its designated repair enzyme-The spore photoproduct lyase.DNA Repair (Amst). 2017 May;53:31-42. doi: 10.1016/j.dnarep.2016.11.005. Epub 2017 Mar 6. DNA Repair (Amst). 2017. PMID: 28320593 Free PMC article.
References
-
- Donnellan JE, Jr, Setlow RB. Thymine photoproducts but not thymine dimers found in ultraviolet-irradiated bacterial spores. Science. 1965;149:308–310. - PubMed
-
- Varghese AJ, Wang SY. Ultraviolet irradiation of DNA in vitro and in vivo produces a third thymine-derived product. Science. 1967;156:955–957. - PubMed
-
- Smith KC, Yoshikawa H. Variation in the photochemical reactivity of thymine in the DNA of B. subtilis spores, vegetative cells and spores germinated in chloramphenicol. Photochem. Photobiol. 1966;5:777–786. - PubMed
-
- Varghese AJ. Photochemistry of thymidine as a thin solid film. Photochem. Photobiol. 1971;13:357–364.
Publication types
MeSH terms
Substances
Grants and funding
LinkOut - more resources
Full Text Sources
Other Literature Sources
Miscellaneous