Synaptic-like vesicles and candidate transduction channels in mechanosensory terminals
- PMID: 26179025
- PMCID: PMC4523322
- DOI: 10.1111/joa.12337
Synaptic-like vesicles and candidate transduction channels in mechanosensory terminals
Abstract
This article summarises progress to date over an exciting and very enjoyable first 15 years of collaboration with Bob Banks. Our collaboration began when I contacted him with (to me) an unexpected observation that a dye used to mark recycling synaptic vesicle membrane at efferent terminals also labelled muscle spindle afferent terminals. This observation led to the re-discovery of a system of small clear vesicles present in all vertebrate primary mechanosensory nerve terminals. These synaptic-like vesicles (SLVs) have been, and continue to be, the major focus of our work. This article describes our characterisation of the properties and functional significance of these SLVs, combining our complementary skills: Bob's technical expertise and encyclopaedic knowledge of mechanosensation with my experience of synaptic vesicles and the development of the styryl pyridinium dyes, of which the most widely used is FM1-43. On the way we have found that SLVs seem to be part of a constitutive glutamate secretory system necessary to maintain the stretch-sensitivity of spindle endings. The glutamate activates a highly unusual glutamate receptor linked to phospholipase D activation, which we have termed the PLD-mGluR. It has a totally distinct pharmacology first described in the hippocampus nearly 20 years ago but, like the SLVs that were first described over 50 years ago, has since been little researched. Yet, our evidence and literature searches suggest this glutamate/SLV/PLD-mGluR system is a ubiquitous feature of mechanosensory endings and, at least for spindles, is essential for maintaining mechanosensory function. This article summarises how this system integrates with the classical model of mechanosensitive channels in spindles and other mechanosensory nerve terminals, including hair follicle afferents and baroreceptors controlling blood pressure. Finally, in this time when there is an imperative to show translational relevance, I describe how this fascinating system might actually be a useful therapeutic drug target for clinical conditions such as hypertension and muscle spasticity. This has been a fascinating 15-year journey in collaboration with Bob who, as well as having an astute scientific mind, is also a great enthusiast, motivator and friend. I hope this exciting and enjoyable journey will continue well into the future.
Keywords: baroreceptors; glutamate; lanceolate endings; mechanosensory terminal; metabotropic glutamate receptor; muscle spindle; synaptic-like vesicles.
© 2015 The Author. Journal of Anatomy published by John Wiley & Sons Ltd on behalf of Anatomical Society.
Figures
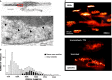
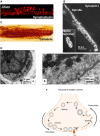
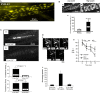
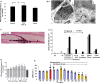
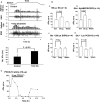
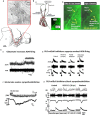
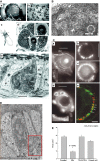
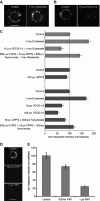
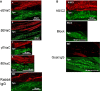
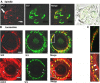
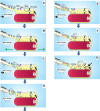
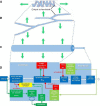
Similar articles
-
The atypical 'hippocampal' glutamate receptor coupled to phospholipase D that controls stretch-sensitivity in primary mechanosensory nerve endings is homomeric purely metabotropic GluK2.Exp Physiol. 2024 Jan;109(1):81-99. doi: 10.1113/EP090761. Epub 2023 Sep 1. Exp Physiol. 2024. PMID: 37656490 Free PMC article. Review.
-
Glutamatergic modulation of synaptic-like vesicle recycling in mechanosensory lanceolate nerve terminals of mammalian hair follicles.J Physiol. 2013 May 15;591(10):2523-40. doi: 10.1113/jphysiol.2012.243659. Epub 2013 Feb 25. J Physiol. 2013. PMID: 23440964 Free PMC article.
-
Modulating mechanosensory afferent excitability by an atypical mGluR.J Anat. 2015 Aug;227(2):214-20. doi: 10.1111/joa.12319. Epub 2015 Jun 5. J Anat. 2015. PMID: 26053109 Free PMC article. Review.
-
Autogenic modulation of mechanoreceptor excitability by glutamate release from synaptic-like vesicles: evidence from the rat muscle spindle primary sensory ending.J Physiol. 2005 Jan 15;562(Pt 2):381-94. doi: 10.1113/jphysiol.2004.074799. Epub 2004 Nov 4. J Physiol. 2005. PMID: 15528245 Free PMC article.
-
Evidence for activity-dependent modulation of sensory-terminal excitability in spindles by glutamate release from synaptic-like vesicles.Adv Exp Med Biol. 2002;508:13-8. doi: 10.1007/978-1-4615-0713-0_2. Adv Exp Med Biol. 2002. PMID: 12171103
Cited by
-
Experimental Physiology special issue: 'Mechanotransduction, muscle spindles and proprioception'.Exp Physiol. 2024 Jan;109(1):1-5. doi: 10.1113/EP091431. Epub 2023 Dec 31. Exp Physiol. 2024. PMID: 38160398 Free PMC article. No abstract available.
-
Acetylcholine receptors in the equatorial region of intrafusal muscle fibres modulate mouse muscle spindle sensitivity.J Physiol. 2019 Apr;597(7):1993-2006. doi: 10.1113/JP277139. Epub 2019 Feb 13. J Physiol. 2019. PMID: 30673133 Free PMC article.
-
The Lamellar Cells of Vertebrate Meissner and Pacinian Corpuscles: Development, Characterization, and Functions.Front Neurosci. 2022 Mar 9;16:790130. doi: 10.3389/fnins.2022.790130. eCollection 2022. Front Neurosci. 2022. PMID: 35356056 Free PMC article.
-
The atypical 'hippocampal' glutamate receptor coupled to phospholipase D that controls stretch-sensitivity in primary mechanosensory nerve endings is homomeric purely metabotropic GluK2.Exp Physiol. 2024 Jan;109(1):81-99. doi: 10.1113/EP090761. Epub 2023 Sep 1. Exp Physiol. 2024. PMID: 37656490 Free PMC article. Review.
-
Generating intrafusal skeletal muscle fibres in vitro: Current state of the art and future challenges.J Tissue Eng. 2020 Dec 29;11:2041731420985205. doi: 10.1177/2041731420985205. eCollection 2020 Jan-Dec. J Tissue Eng. 2020. PMID: 34956586 Free PMC article. Review.
References
-
- Aguado F, Majó G, Ruiz-Montasell B, et al. Syntaxin 1A and 1B display distinct distribution patterns in the rat peripheral nervous system. Neuroscience. 1999;88:437–446. - PubMed
-
- Akoev GN, Alekseev NP, Krylov BV. Mechanoreceptors: Their Functional Organisation. Berlin: Springer; 1988.
-
- Albani-Torregrossa S, Attucci S, Marinozzi M, et al. Antagonist pharmacology of metabotropic glutamate receptors coupled to phospholipase D activation in adult rat hippocampus: focus on (2R,1’S,2’R,3’S)-2-(2′-carboxy-3′-phenylcyclopropyl)glycine versus 3, 5-dihydroxyphenylglycine. Mol Pharmacol. 1999;55:699–707. - PubMed
-
- Arnadóttir J, Chalfie M. Eukaryotic mechanosensitive channels. Ann Rev Biophy. 2010;39:111–137. - PubMed
-
- Banks RW, Bewick GS, Reid B, et al. Evidence for activity-dependent modulation of sensory-terminal excitability in spindles by glutamate release from synaptic-like vesicles. Adv Exp Med Biol. 2002;508:13–18. - PubMed
Publication types
MeSH terms
Substances
Grants and funding
LinkOut - more resources
Full Text Sources
Other Literature Sources