Hippocampal sharp wave-ripple: A cognitive biomarker for episodic memory and planning
- PMID: 26135716
- PMCID: PMC4648295
- DOI: 10.1002/hipo.22488
Hippocampal sharp wave-ripple: A cognitive biomarker for episodic memory and planning
Abstract
Sharp wave ripples (SPW-Rs) represent the most synchronous population pattern in the mammalian brain. Their excitatory output affects a wide area of the cortex and several subcortical nuclei. SPW-Rs occur during "off-line" states of the brain, associated with consummatory behaviors and non-REM sleep, and are influenced by numerous neurotransmitters and neuromodulators. They arise from the excitatory recurrent system of the CA3 region and the SPW-induced excitation brings about a fast network oscillation (ripple) in CA1. The spike content of SPW-Rs is temporally and spatially coordinated by a consortium of interneurons to replay fragments of waking neuronal sequences in a compressed format. SPW-Rs assist in transferring this compressed hippocampal representation to distributed circuits to support memory consolidation; selective disruption of SPW-Rs interferes with memory. Recently acquired and pre-existing information are combined during SPW-R replay to influence decisions, plan actions and, potentially, allow for creative thoughts. In addition to the widely studied contribution to memory, SPW-Rs may also affect endocrine function via activation of hypothalamic circuits. Alteration of the physiological mechanisms supporting SPW-Rs leads to their pathological conversion, "p-ripples," which are a marker of epileptogenic tissue and can be observed in rodent models of schizophrenia and Alzheimer's Disease. Mechanisms for SPW-R genesis and function are discussed in this review.
Keywords: epilepsy; imagining; learning; memory; planning.
© 2015 The Authors Hippocampus Published by Wiley Periodicals, Inc.
Figures
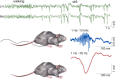
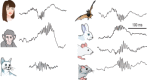
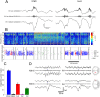
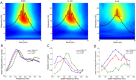
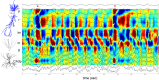
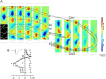
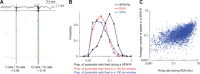
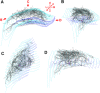
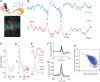
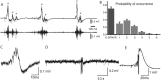
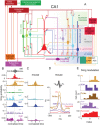
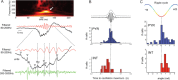
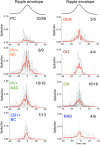
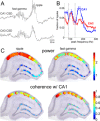
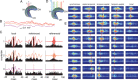
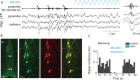
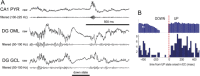
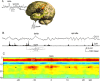
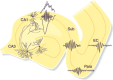
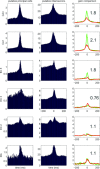
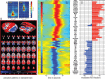
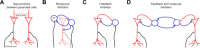
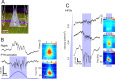
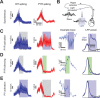
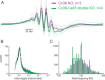
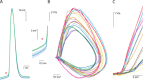
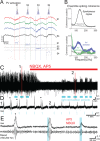
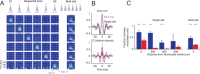
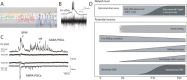
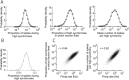
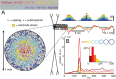
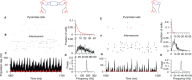
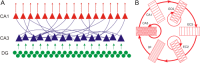
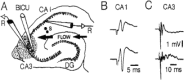
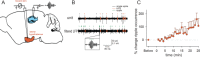
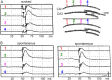
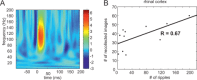
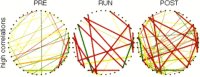
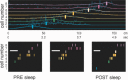
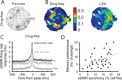
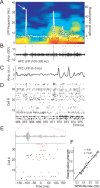
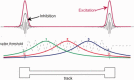
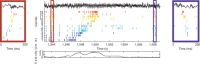
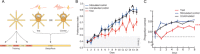
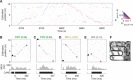
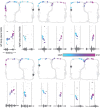
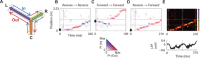
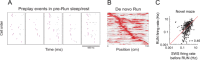
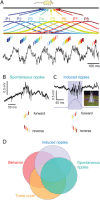
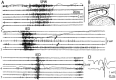
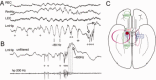
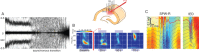
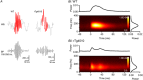
Similar articles
-
A Unified Dynamic Model for Learning, Replay, and Sharp-Wave/Ripples.J Neurosci. 2015 Dec 9;35(49):16236-58. doi: 10.1523/JNEUROSCI.3977-14.2015. J Neurosci. 2015. PMID: 26658873 Free PMC article.
-
Most hippocampal CA1 pyramidal cells in rabbits increase firing during awake sharp-wave ripples and some do so in response to external stimulation and theta.J Neurophysiol. 2020 May 1;123(5):1671-1681. doi: 10.1152/jn.00056.2020. Epub 2020 Mar 25. J Neurophysiol. 2020. PMID: 32208887
-
Impairment of Sharp-Wave Ripples in a Murine Model of Dravet Syndrome.J Neurosci. 2019 Nov 13;39(46):9251-9260. doi: 10.1523/JNEUROSCI.0890-19.2019. Epub 2019 Sep 19. J Neurosci. 2019. PMID: 31537705 Free PMC article.
-
Exploring Ripple Waves in the Human Brain.Clin EEG Neurosci. 2023 Nov;54(6):594-600. doi: 10.1177/15500594211034371. Epub 2021 Jul 21. Clin EEG Neurosci. 2023. PMID: 34287087 Review.
-
Hippocampal ripples as a mode of communication with cortical and subcortical areas.Hippocampus. 2020 Jan;30(1):39-49. doi: 10.1002/hipo.22997. Epub 2018 Nov 13. Hippocampus. 2020. PMID: 30069976 Review.
Cited by
-
Decoding episodic autobiographical memory in naturalistic virtual reality.Sci Rep. 2024 Oct 27;14(1):25639. doi: 10.1038/s41598-024-76944-3. Sci Rep. 2024. PMID: 39463396 Free PMC article.
-
Coordinated Interaction between Hippocampal Sharp-Wave Ripples and Anterior Cingulate Unit Activity.J Neurosci. 2016 Oct 12;36(41):10663-10672. doi: 10.1523/JNEUROSCI.1042-16.2016. J Neurosci. 2016. PMID: 27733616 Free PMC article.
-
Basal Forebrain Cholinergic Innervation Induces Depression-Like Behaviors Through Ventral Subiculum Hyperactivation.Neurosci Bull. 2023 Apr;39(4):617-630. doi: 10.1007/s12264-022-00962-2. Epub 2022 Nov 7. Neurosci Bull. 2023. PMID: 36342657 Free PMC article.
-
Sharp-wave ripple doublets induce complex dendritic spikes in parvalbumin interneurons in vivo.Nat Commun. 2022 Nov 7;13(1):6715. doi: 10.1038/s41467-022-34520-1. Nat Commun. 2022. PMID: 36344570 Free PMC article.
-
Construction of ThermoMaze.Bio Protoc. 2024 Aug 5;14(15):e5044. doi: 10.21769/BioProtoc.5044. eCollection 2024 Aug 5. Bio Protoc. 2024. PMID: 39131192 Free PMC article.
References
-
- Abeles M, Bergman H, Margalit E, Vaadia E. 1993. Spatiotemporal firing patterns in the frontal cortex of behaving monkeys. J Neurophysiol 70:1629–1638. - PubMed
-
- Abraham WC, Gustafsson B, Wigstrom H. 1986. Single high strength afferent volleys can produce long‐term potentiation in the hippocampus in vitro. Neurosci Lett 70:217–222. - PubMed
-
- Adey WR, Kado RT, Rhodes JM. 1963. Sleep: Cortical and subcortical recordings in the chimpanzee. Science 141:932–933. - PubMed
Publication types
MeSH terms
Substances
Grants and funding
LinkOut - more resources
Full Text Sources
Other Literature Sources
Miscellaneous