Cortical instability drives periodic supracellular actin pattern formation in epithelial tubes
- PMID: 26077909
- PMCID: PMC4507253
- DOI: 10.1073/pnas.1504762112
Cortical instability drives periodic supracellular actin pattern formation in epithelial tubes
Abstract
An essential question of morphogenesis is how patterns arise without preexisting positional information, as inspired by Turing. In the past few years, cytoskeletal flows in the cell cortex have been identified as a key mechanism of molecular patterning at the subcellular level. Theoretical and in vitro studies have suggested that biological polymers such as actomyosin gels have the property to self-organize, but the applicability of this concept in an in vivo setting remains unclear. Here, we report that the regular spacing pattern of supracellular actin rings in the Drosophila tracheal tubule is governed by a self-organizing principle. We propose a simple biophysical model where pattern formation arises from the interplay of myosin contractility and actin turnover. We validate the hypotheses of the model using photobleaching experiments and report that the formation of actin rings is contractility dependent. Moreover, genetic and pharmacological perturbations of the physical properties of the actomyosin gel modify the spacing of the pattern, as the model predicted. In addition, our model posited a role of cortical friction in stabilizing the spacing pattern of actin rings. Consistently, genetic depletion of apical extracellular matrix caused strikingly dynamic movements of actin rings, mirroring our model prediction of a transition from steady to chaotic actin patterns at low cortical friction. Our results therefore demonstrate quantitatively that a hydrodynamical instability of the actin cortex can trigger regular pattern formation and drive morphogenesis in an in vivo setting.
Keywords: Drosophila; actomyosin; biological tubes; biophysics; pattern formation.
Conflict of interest statement
The authors declare no conflict of interest.
Figures
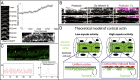
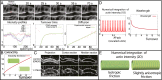
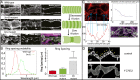
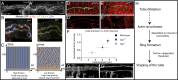
Comment in
-
Three-ring circus without a ringmaster: Self-organization of supracellular actin ring patterns during epithelial morphogenesis.Proc Natl Acad Sci U S A. 2015 Jul 14;112(28):8521-2. doi: 10.1073/pnas.1510614112. Epub 2015 Jul 6. Proc Natl Acad Sci U S A. 2015. PMID: 26150495 Free PMC article. No abstract available.
Similar articles
-
Self-organizing actomyosin patterns on the cell cortex at epithelial cell-cell junctions.Biophys J. 2014 Dec 2;107(11):2652-61. doi: 10.1016/j.bpj.2014.10.045. Epub 2014 Dec 2. Biophys J. 2014. PMID: 25468344 Free PMC article.
-
Emergent mechanics of actomyosin drive punctuated contractions and shape network morphology in the cell cortex.PLoS Comput Biol. 2018 Sep 17;14(9):e1006344. doi: 10.1371/journal.pcbi.1006344. eCollection 2018 Sep. PLoS Comput Biol. 2018. PMID: 30222728 Free PMC article.
-
Cross-linker-mediated regulation of actin network organization controls tissue morphogenesis.J Cell Biol. 2019 Aug 5;218(8):2743-2761. doi: 10.1083/jcb.201811127. Epub 2019 Jun 28. J Cell Biol. 2019. PMID: 31253650 Free PMC article.
-
Contractile and expansive actin networks in Drosophila: Developmental cell biology controlled by network polarization and higher-order interactions.Curr Top Dev Biol. 2023;154:99-129. doi: 10.1016/bs.ctdb.2023.02.005. Epub 2023 Mar 14. Curr Top Dev Biol. 2023. PMID: 37100525 Review.
-
Patterns in space: coordinating adhesion and actomyosin contractility at E-cadherin junctions.Cell Commun Adhes. 2013 Dec;20(6):201-12. doi: 10.3109/15419061.2013.856889. Epub 2013 Nov 8. Cell Commun Adhes. 2013. PMID: 24205985 Review.
Cited by
-
Myosin turnover controls actomyosin contractile instability.Proc Natl Acad Sci U S A. 2022 Oct 25;119(43):e2211431119. doi: 10.1073/pnas.2211431119. Epub 2022 Oct 20. Proc Natl Acad Sci U S A. 2022. PMID: 36264833 Free PMC article.
-
Actin crosslinking by α-actinin averts viscous dissipation of myosin force transmission in stress fibers.iScience. 2023 Feb 1;26(3):106090. doi: 10.1016/j.isci.2023.106090. eCollection 2023 Mar 17. iScience. 2023. PMID: 36852278 Free PMC article.
-
Anisotropic expansion of hepatocyte lumina enforced by apical bulkheads.J Cell Biol. 2021 Oct 4;220(10):e202103003. doi: 10.1083/jcb.202103003. Epub 2021 Jul 30. J Cell Biol. 2021. PMID: 34328499 Free PMC article.
-
Cell Surface Mechanochemistry and the Determinants of Bleb Formation, Healing, and Travel Velocity.Biophys J. 2016 Apr 12;110(7):1636-1647. doi: 10.1016/j.bpj.2016.03.008. Biophys J. 2016. PMID: 27074688 Free PMC article.
-
Structural Redundancy in Supracellular Actomyosin Networks Enables Robust Tissue Folding.Dev Cell. 2019 Sep 9;50(5):586-598.e3. doi: 10.1016/j.devcel.2019.06.015. Epub 2019 Jul 25. Dev Cell. 2019. PMID: 31353314 Free PMC article.
References
-
- Keller EF, Segel LA. Model for chemotaxis. J Theor Biol. 1971;30(2):225–234. - PubMed
-
- Howard J, Grill SW, Bois JS. Turing’s next steps: The mechanochemical basis of morphogenesis. Nat Rev Mol Cell Biol. 2011;12(6):392–398. - PubMed
-
- Maini PK. The impact of Turing’s work on pattern formation in biology. Math Today. 2004;40(4):140–141.
Publication types
MeSH terms
Substances
LinkOut - more resources
Full Text Sources
Other Literature Sources
Molecular Biology Databases
Research Materials
Miscellaneous