A Neural Circuit That Controls Cortical State, Plasticity, and the Gain of Sensory Responses in Mouse
- PMID: 25948638
- PMCID: PMC4500789
- DOI: 10.1101/sqb.2014.79.024927
A Neural Circuit That Controls Cortical State, Plasticity, and the Gain of Sensory Responses in Mouse
Abstract
Neurons in the visual cortex were first found to be exquisitely selective for particular properties of visual stimuli in anesthetized animals, including mice. Studies of alert mice in an apparatus that allowed them to stand or run revealed that locomotion causes a change in cortical state that dramatically increases the magnitude of responses in neurons of the visual cortex without altering selectivity, effectively changing the gain of sensory responses. Locomotion also dramatically enhances adult plasticity in the recovery from long-term visual deprivation. We have studied the elements and operation of the neural circuit responsible for the enhancement of activity and shown that it enhances plasticity even in mice not free to run. The circuit consists of projections ascending from the midbrain locomotor region (MLR) to the basal forebrain, activating cholinergic and perhaps other projections to excite inhibitory interneurons expressing vasoactive intestinal peptide (VIP) in the visual cortex. VIP cells activated by locomotion inhibit interneurons that express somatostatin (SST), thereby disinhibiting the excitatory principal neurons and allowing them to respond more strongly to effective visual stimuli. These findings reveal in alert animals how the ascending reticular activating system described in anesthetized animals 50 years ago operates to control cortical state.
Copyright © 2014 Cold Spring Harbor Laboratory Press; all rights reserved.
Figures
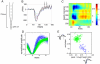
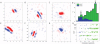
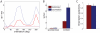
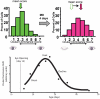
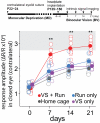
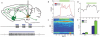
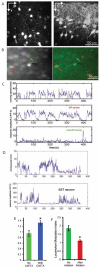
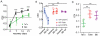
Similar articles
-
A cortical disinhibitory circuit for enhancing adult plasticity.Elife. 2015 Jan 27;4:e05558. doi: 10.7554/eLife.05558. Elife. 2015. PMID: 25626167 Free PMC article.
-
Identification of a brainstem circuit regulating visual cortical state in parallel with locomotion.Neuron. 2014 Jul 16;83(2):455-466. doi: 10.1016/j.neuron.2014.06.031. Neuron. 2014. PMID: 25033185 Free PMC article.
-
Activation of Somatostatin Interneurons by Nicotinic Modulator Lypd6 Enhances Plasticity and Functional Recovery in the Adult Mouse Visual Cortex.J Neurosci. 2020 Jul 1;40(27):5214-5227. doi: 10.1523/JNEUROSCI.1373-19.2020. Epub 2020 May 28. J Neurosci. 2020. PMID: 32467358 Free PMC article.
-
Initiation of locomotion in lampreys.Brain Res Rev. 2008 Jan;57(1):172-82. doi: 10.1016/j.brainresrev.2007.07.016. Epub 2007 Aug 22. Brain Res Rev. 2008. PMID: 17916380 Review.
-
Cortical inhibitory interneurons control sensory processing.Curr Opin Neurobiol. 2017 Oct;46:200-207. doi: 10.1016/j.conb.2017.08.018. Epub 2017 Sep 20. Curr Opin Neurobiol. 2017. PMID: 28938181 Free PMC article. Review.
Cited by
-
Brain-Wide Maps of Synaptic Input to Cortical Interneurons.J Neurosci. 2016 Apr 6;36(14):4000-9. doi: 10.1523/JNEUROSCI.3967-15.2016. J Neurosci. 2016. PMID: 27053207 Free PMC article.
-
Reducing GABAA-mediated inhibition improves forelimb motor function after focal cortical stroke in mice.Sci Rep. 2016 Nov 29;6:37823. doi: 10.1038/srep37823. Sci Rep. 2016. PMID: 27897203 Free PMC article.
-
Moderate physical activity alters the estimation of time, but not space.Front Psychol. 2022 Oct 5;13:1004504. doi: 10.3389/fpsyg.2022.1004504. eCollection 2022. Front Psychol. 2022. PMID: 36275247 Free PMC article.
-
Antioxidants Prevent the Effects of Physical Exercise on Visual Cortical Plasticity.Cells. 2022 Dec 22;12(1):48. doi: 10.3390/cells12010048. Cells. 2022. PMID: 36611842 Free PMC article.
-
A Synthetic Agonist to Vasoactive Intestinal Peptide Receptor-2 Induces Regulatory T Cell Neuroprotective Activities in Models of Parkinson's Disease.Front Cell Neurosci. 2019 Sep 18;13:421. doi: 10.3389/fncel.2019.00421. eCollection 2019. Front Cell Neurosci. 2019. PMID: 31619964 Free PMC article.
References
Publication types
MeSH terms
Grants and funding
LinkOut - more resources
Full Text Sources
Other Literature Sources