Nanotopographical Surfaces for Stem Cell Fate Control: Engineering Mechanobiology from the Bottom
- PMID: 25883674
- PMCID: PMC4394389
- DOI: 10.1016/j.nantod.2014.12.002
Nanotopographical Surfaces for Stem Cell Fate Control: Engineering Mechanobiology from the Bottom
Abstract
During embryogenesis and tissue maintenance and repair in an adult organism, a myriad of stem cells are regulated by their surrounding extracellular matrix (ECM) enriched with tissue/organ-specific nanoscale topographical cues to adopt different fates and functions. Attributed to their capability of self-renewal and differentiation into most types of somatic cells, stem cells also hold tremendous promise for regenerative medicine and drug screening. However, a major challenge remains as to achieve fate control of stem cells in vitro with high specificity and yield. Recent exciting advances in nanotechnology and materials science have enabled versatile, robust, and large-scale stem cell engineering in vitro through developments of synthetic nanotopographical surfaces mimicking topological features of stem cell niches. In addition to generating new insights for stem cell biology and embryonic development, this effort opens up unlimited opportunities for innovations in stem cell-based applications. This review is therefore to provide a summary of recent progress along this research direction, with perspectives focusing on emerging methods for generating nanotopographical surfaces and their applications in stem cell research. Furthermore, we provide a review of classical as well as emerging cellular mechano-sensing and -transduction mechanisms underlying stem cell nanotopography sensitivity and also give some hypotheses in regard to how a multitude of signaling events in cellular mechanotransduction may converge and be integrated into core pathways controlling stem cell fate in response to extracellular nanotopography.
Keywords: Biomaterials; Mechanobiology; Nanotopography; Stem cell; Tissue engineering and regenerative medicine.
Figures
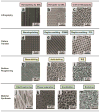
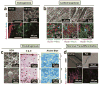
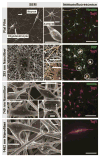
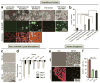
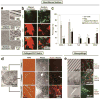
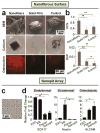
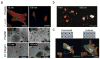
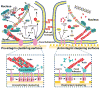
Similar articles
-
An update of nanotopographical surfaces in modulating stem cell fate: a narrative review.Biomater Transl. 2022 Mar 28;3(1):55-64. doi: 10.12336/biomatertransl.2022.01.006. eCollection 2022. Biomater Transl. 2022. PMID: 35837345 Free PMC article. Review.
-
Modulation of human multipotent and pluripotent stem cells using surface nanotopographies and surface-immobilised bioactive signals: A review.Acta Biomater. 2016 Nov;45:31-59. doi: 10.1016/j.actbio.2016.08.054. Epub 2016 Sep 3. Acta Biomater. 2016. PMID: 27596488 Review.
-
Control of stem cell fate by engineering their micro and nanoenvironment.World J Stem Cells. 2015 Jan 26;7(1):37-50. doi: 10.4252/wjsc.v7.i1.37. World J Stem Cells. 2015. PMID: 25621104 Free PMC article. Review.
-
Nanotechnology in the regulation of stem cell behavior.Sci Technol Adv Mater. 2013 Oct 11;14(5):054401. doi: 10.1088/1468-6996/14/5/054401. eCollection 2013 Oct. Sci Technol Adv Mater. 2013. PMID: 27877605 Free PMC article. Review.
-
Response of mesenchymal stem cells to surface topography of scaffolds and the underlying mechanisms.J Mater Chem B. 2023 Mar 22;11(12):2550-2567. doi: 10.1039/d2tb01875f. J Mater Chem B. 2023. PMID: 36852826 Review.
Cited by
-
Nondestructive Real-Time Monitoring of Enhanced Stem Cell Differentiation Using a Graphene-Au Hybrid Nanoelectrode Array.Adv Mater. 2018 Sep;30(39):e1802762. doi: 10.1002/adma.201802762. Epub 2018 Aug 2. Adv Mater. 2018. PMID: 30073706 Free PMC article.
-
DNA/Magnetic Nanoparticles Composite to Attenuate Glass Surface Nanotopography for Enhanced Mesenchymal Stem Cell Differentiation.Polymers (Basel). 2022 Jan 17;14(2):344. doi: 10.3390/polym14020344. Polymers (Basel). 2022. PMID: 35054750 Free PMC article.
-
Decoupling the effects of nanopore size and surface roughness on the attachment, spreading and differentiation of bone marrow-derived stem cells.Biomaterials. 2020 Jul;248:120014. doi: 10.1016/j.biomaterials.2020.120014. Epub 2020 Mar 31. Biomaterials. 2020. PMID: 32276040 Free PMC article.
-
3D Printed, Microgroove Pattern-Driven Generation of Oriented Ligamentous Architectures.Int J Mol Sci. 2017 Sep 8;18(9):1927. doi: 10.3390/ijms18091927. Int J Mol Sci. 2017. PMID: 28885543 Free PMC article.
-
Biomaterials to enhance stem cell transplantation.Cell Stem Cell. 2022 May 5;29(5):692-721. doi: 10.1016/j.stem.2022.04.002. Epub 2022 Apr 27. Cell Stem Cell. 2022. PMID: 35483364 Free PMC article. Review.
References
-
- Daley GQ, Scadden DT. Cell. 2008;132:544–548. - PubMed
-
- Nishikawa SI, Jakt LM, Era T. Nat Rev Mol Cell Biol. 2007;8:502–507. - PubMed
-
- Jopling C, Boue S, Belmonte JCI. Nat Rev Mol Cell Biol. 2011;12:79–89. - PubMed
-
- Singec I, Jandial R, Crain A, Nikkhah G, Snyder EY. Annu Rev Cell Dev Biol. 2007;58:313–328. - PubMed
Grants and funding
LinkOut - more resources
Full Text Sources
Other Literature Sources