Protein design: Past, present, and future
- PMID: 25784145
- PMCID: PMC4856012
- DOI: 10.1002/bip.22639
Protein design: Past, present, and future
Abstract
Building on the pioneering work of Ho and DeGrado (J Am Chem Soc 1987, 109, 6751-6758) in the late 1980s, protein design approaches have revealed many fundamental features of protein structure and stability. We are now in the era that the early work presaged - the design of new proteins with practical applications and uses. Here we briefly survey some past milestones in protein design, in addition to highlighting recent progress and future aspirations.
Keywords: computation; nanotechnology; protein design; review.
© 2015 Wiley Periodicals, Inc.
Figures
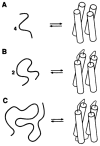
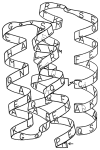
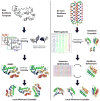
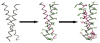
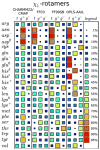
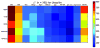
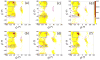
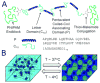
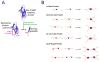
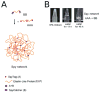
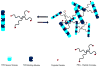
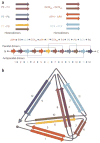
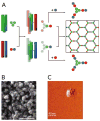
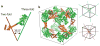
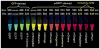
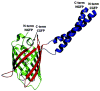
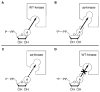
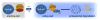
Similar articles
-
Engineering protein and peptide building blocks for nanotechnology.J Nanosci Nanotechnol. 2007 Feb;7(2):387-401. doi: 10.1166/jnn.2007.153. J Nanosci Nanotechnol. 2007. PMID: 17450770 Review.
-
Recent trends in biocatalysis engineering.Bioresour Technol. 2012 Jul;115:48-57. doi: 10.1016/j.biortech.2011.12.050. Epub 2011 Dec 24. Bioresour Technol. 2012. PMID: 22424920 Review.
-
Review: protein design--where we were, where we are, where we're going.J Struct Biol. 2001 May-Jun;134(2-3):269-81. doi: 10.1006/jsbi.2001.4349. J Struct Biol. 2001. PMID: 11551185 Review.
-
Protein Design for Nanostructural Engineering: Concluding Remarks and Future Directions.Adv Exp Med Biol. 2016;940:281-284. doi: 10.1007/978-3-319-39196-0_12. Adv Exp Med Biol. 2016. PMID: 27677517 Review.
-
Progress in computational protein design.Curr Opin Biotechnol. 2007 Aug;18(4):305-11. doi: 10.1016/j.copbio.2007.04.009. Epub 2007 Jul 20. Curr Opin Biotechnol. 2007. PMID: 17644370 Free PMC article. Review.
Cited by
-
ISAMBARD: an open-source computational environment for biomolecular analysis, modelling and design.Bioinformatics. 2017 Oct 1;33(19):3043-3050. doi: 10.1093/bioinformatics/btx352. Bioinformatics. 2017. PMID: 28582565 Free PMC article.
-
Exo-chirality of the α-helix.Nat Commun. 2024 Aug 14;15(1):6987. doi: 10.1038/s41467-024-51072-8. Nat Commun. 2024. PMID: 39143054 Free PMC article.
-
Peptide Assembly Directed and Quantified Using Megadalton DNA Nanostructures.ACS Nano. 2019 Sep 24;13(9):9927-9935. doi: 10.1021/acsnano.9b04251. Epub 2019 Aug 8. ACS Nano. 2019. PMID: 31381314 Free PMC article.
-
The coming of age of de novo protein design.Nature. 2016 Sep 15;537(7620):320-7. doi: 10.1038/nature19946. Nature. 2016. PMID: 27629638 Review.
-
The G protein coupled receptor CXCR4 designed by the QTY code becomes more hydrophilic and retains cell signaling activity.Sci Rep. 2020 Dec 7;10(1):21371. doi: 10.1038/s41598-020-77659-x. Sci Rep. 2020. PMID: 33288780 Free PMC article.
References
Publication types
MeSH terms
Grants and funding
LinkOut - more resources
Full Text Sources
Other Literature Sources
Miscellaneous