FGF22 signaling regulates synapse formation during post-injury remodeling of the spinal cord
- PMID: 25766255
- PMCID: PMC4426482
- DOI: 10.15252/embj.201490578
FGF22 signaling regulates synapse formation during post-injury remodeling of the spinal cord
Abstract
The remodeling of axonal circuits after injury requires the formation of new synaptic contacts to enable functional recovery. Which molecular signals initiate such axonal and synaptic reorganisation in the adult central nervous system is currently unknown. Here, we identify FGF22 as a key regulator of circuit remodeling in the injured spinal cord. We show that FGF22 is produced by spinal relay neurons, while its main receptors FGFR1 and FGFR2 are expressed by cortical projection neurons. FGF22 deficiency or the targeted deletion of FGFR1 and FGFR2 in the hindlimb motor cortex limits the formation of new synapses between corticospinal collaterals and relay neurons, delays their molecular maturation, and impedes functional recovery in a mouse model of spinal cord injury. These results establish FGF22 as a synaptogenic mediator in the adult nervous system and a crucial regulator of synapse formation and maturation during post-injury remodeling in the spinal cord.
Keywords: axonal remodeling; fibroblast growth factor; functional recovery; spinal cord injury; synapse formation.
© 2015 The Authors.
Figures
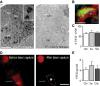
In situ hybridization of FGF22 mRNA in the spinal cord of FGF22-competent (left panel) and FGF22-deficient (right panel) mice (DH: dorsal horn; VH: ventral horn). Scale bar equals 200 μm (10 μm in inset).
In situ hybridization of a section of the cervical spinal cord at level C4/C5 showing presence of FGF22 mRNA in a long propriospinal neuron (LPSN) retrogradely traced from T12 (LPSN: green; FGF22 mRNA: red; NeuroTrace 435/455: blue). Scale bar equals 20 μm.
Quantification of the percentage of LPSN showing a FGF22 in situ signal in unlesioned mice (‘Ctrl’) and in mice at 3 (‘3w’) and 12 (‘12w’) weeks after spinal cord injury (n > 30 LPSN from 2 to 3 animals per group). Mean ± SEM. No significant differences between the groups were detected (ANOVA followed by Tukey tests).
Images of LPSN (red) before (left panel) and after (right panel) laser microdissection of a single neuron (arrow, asterisk and dotted line in right panel indicate previous location of the microdissected neuron). Scale bar equals 40 μm.
Quantification of the single-cell PCR analysis of FGF22 mRNA expression in LPSN dissected from unlesioned mice (‘Ctrl’) and from mice at 3 (‘3w’) and 12 (‘12w’) weeks after spinal cord injury (n = 7–8 LPSN per group). Mean ± SEM. No significant differences between the groups were detected (ANOVA followed by Tukey tests).

Schematic representation of CST detour circuit formation following a mid-thoracic dorsal bilateral hemisection of the spinal cord.
Confocal images of hindlimb CST collaterals exiting the main CST tract (arrows) in the cervical spinal cord 3 weeks following a T8 dorsal bilateral hemisection in FGF22-competent (left panel) and FGF22-deficient (right panel) mice. Scale bar equals 40 μm.
Quantification of the number of exiting hindlimb CST collaterals per labeled hindlimb CST fiber at 3 weeks following T8 dorsal bilateral hemisection in FGF22-competent and FGF22-deficient mice (n = 8 animals per group). Mean ± SEM. No significant differences between the groups were detected (unpaired two-tailed t-test).
Confocal images showing putative synaptic boutons (arrows) on newly formed cervical hindlimb CST collaterals at 3 weeks following spinal cord injury in FGF22-competent (left panel) and FGF22-deficient (right panel) mice. Scale bar equals 20 μm.
Quantification of bouton density on newly formed cervical hindlimb CST collaterals in FGF22-competent and FGF22-deficient mice (n = 8 animals per group) at 3 weeks after injury. Mean ± SEM. *P = 0.0244 (unpaired two-tailed t-test).
3D Rendering of a confocal image stack that illustrates putative synaptic contacts between CST collaterals (green) and LPSN (red) counterstained with NeuroTrace 435/455 (blue). Scale bar equals 30 μm.
Quantification of the percentage of LPSN contacted by cervical hindlimb CST collaterals in FGF22-competent and FGF22-deficient mice at 3 weeks after injury (n = 8 animals per group). Mean ± SEM. ***P = 0.0001 (unpaired two-tailed t-test).
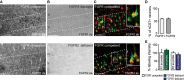
In situ hybridization of FGFR1 (top) and FGFR2 (bottom) mRNA in FGFR-competent mice. Scale bar equals 200 μm.
In situ hybridization of FGFR1 (top) and FGFR2 (bottom) mRNA in forebrain FGFR1 (top)- and FGFR2 (bottom)-deficient mice. Scale bar equals 200 μm.
Retrograde labeling of CST projection neurons with dextran conjugated with Texas Red® (green) shows that many of these neurons express FGFR1 (red, top) and FGFR2 mRNA (red, bottom; insets in top and bottom panels are twofold magnification of boxed areas). Scale bar equals 100 μm (20 μm in insets).
Quantification of the percentage of CST projection neurons in layer V of the cortex expressing FGFR1 (white bar) and FGFR2 mRNA (gray bar; n = 5 animals per group). Mean ± SEM.
Quantification of the intensity of the in situ signal for FGFR1 mRNA in FGFR2-deficient mice (green bar) and FGFR2 mRNA in FGFR1-deficient mice (blue bar) normalized to the signal intensity measured in the respective FGFR-competent control group (white bars; n = 3 animals per group). Mean ± SEM. No significant differences between the groups were detected (unpaired two-tailed t-tests).
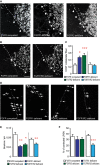
Confocal images of hindlimb CST collaterals exiting the main CST tract (arrows) in the cervical spinal cord 3 weeks following T8 dorsal bilateral hemisection in FGFR-competent (left panel), forebrain FGFR1-deficient (middle panel), and forebrain FGFR2-deficient (right panel) mice. Scale bar equals 40 μm.
Confocal images of hindlimb CST collaterals exiting the main CST tract (arrows) in the cervical spinal cord 3 weeks following T8 dorsal bilateral hemisection in FGFR-competent (left panel) and hindlimb motor cortex FGFR1/FGFR2 double-deficient (right panel) mice. Scale bar equals 40 μm.
Quantification of the number of exiting hindlimb CST collaterals at 3 weeks following T8 dorsal bilateral hemisection in forebrain FGFR single-deficient mice, hindlimb motor cortex FGFR1/FGFR2 double-deficient mice, and the corresponding FGFR-competent control mice (n = 6–15 animals per group). Mean ± SEM. *P < 0.05; ***P < 0.001 (ANOVA followed by Tukey tests for FGFR-competent versus FGFR single-deficient mice). No significant differences were found between FGFR-competent and FGFR1/FGFR2 double-deficient mice (unpaired two-tailed t-tests).
Confocal images showing putative synaptic boutons (arrows) on newly formed cervical hindlimb CST collaterals at 3 weeks following spinal cord injury in FGFR-competent (left panel), forebrain FGFR1-deficient (second panel from left), forebrain FGFR2-deficient (second panel from right), and hindlimb motor cortex FGFR1/FGFR2 double-deficient (right panel) mice. Scale bar equals 20 μm.
Quantification of the bouton density on newly formed cervical hindlimb CST collaterals in FGFR-competent, forebrain single FGFR-deficient, and hindlimb motor cortex FGFR1/FGFR2 double-deficient mice (n = 6–16 animals per group). Mean ± SEM. *P < 0.05, **P < 0.01 (ANOVA followed by Tukey tests in case of multiple group comparisons, e.g. FGFR-competent versus FGFR single-deficient mice). **P = 0.0028 (unpaired two-tailed t-tests for comparisons of FGFR-competent versus FGFR1/FGFR2 double-deficient mice).
Quantification of the percentage of LPSN contacted by hindlimb CST collaterals in FGFR-competent, forebrain single FGFR-deficient, and hindlimb motor cortex FGFR1/FGFR2 double-deficient mice (n = 6–16 animals per group). ***P < 0.0001 (unpaired two-tailed t-tests for comparisons of controls versus FGFR1/FGFR2 double-deficient mice). No significant differences were found between FGFR-competent and FGFR single-deficient mice (ANOVA followed by Tukey tests).
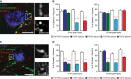
Confocal image of synaptic contacts (arrows) between a CST collateral (green) and a LPSN (blue) that show bassoon immunoreactivity (red). Right images are magnification (two and a half-fold) of the area boxed on the left. Scale bar equals 25 μm.
Quantification of the percentage of boutons on cervical hindlimb CST collaterals that are immunoreactive for bassoon at 3 weeks (left) and 12 weeks (right) after spinal cord injury in FGF22-deficient, forebrain FGFR1-deficient, forebrain FGFR2-deficient and hindlimb motor cortex FGFR1/FGFR2 double-deficient mice compared to the respective FGF22- and FGFR-competent control mice. A minimum of 100 boutons per mouse were evaluated for 3 mice per group. ***P < 0.0001 FGFR-competent versus FGFR1/FGFR2 double-deficient mice at 3 weeks (unpaired two-tailed t-tests), **P = 0.002 FGF-competent versus FGF22 deficient at 3 weeks (unpaired two-tailed t-tests), *P < 0.05 FGFR-competent versus FGFR2 single-deficient mice at 12 weeks (one-way ANOVA followed by Tukey tests), ***P = 0.001 FGFR-competent versus FGFR1/FGFR2 double-deficient mice at 12 weeks (unpaired two-tailed t-tests).
Confocal image of synaptic contacts (arrows) between a CST collateral (green) and a LPSN (blue) that show synapsin I immunoreactivity (red). Right images are magnification (two and a half-fold) of the area boxed on the left. Scale bar equals 25 μm.
Quantification of the percentage of boutons on cervical hindlimb CST collaterals that are immunoreactive for synapsin I at 3 weeks (left) and 12 weeks (right) after spinal cord injury in FGF22-deficient, forebrain FGFR1-deficient, forebrain FGFR2-deficient and hindlimb motor cortex FGFR1/FGFR2 double-deficient mice compared to the respective FGF22 and FGFR-competent control mice. A minimum of 100 boutons per mouse were evaluated for 3 mice per group. ***P < 0.001 FGFR-competent versus FGFR1 and FGFR2 single-deficient mice at 3 weeks (one-way ANOVA followed by Tukey tests). ***P = 0.0001 FGFR-competent mice versus FGFR1/FGFR2 double-deficient mice at 3 weeks (unpaired two-tailed t-tests), P = 0.00004 FGF-competent mice versus FGF22-deficient mice at 3 weeks (unpaired two-tailed t-tests). ***P < 0.001 FGFR-competent versus FGFR2 single-deficient mice (one-way ANOVA followed by Tukey tests). ***P = 0.0006 FGFR-competent mice versus FGFR1/FGFR2 double-deficient mice at 12 weeks (unpaired two-tailed t-tests), *P = 0.027 FGF-competent mice versus FGF22-deficient mice at 12 weeks (unpaired two-tailed t-tests).
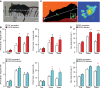
Image of a spinal cord injured mouse performing the irregular ladder rung test that assesses recovery of CST function. Scale bar equals 1 cm.
Quantification of the functional recovery in the ladder rung test (regular walk, left panels; irregular walk, right panels) in FGF22-deficient (top panels, red bars), and hindlimb motor cortex FGFR1/FGFR2 double-deficient (bottom panels, blue bars) mice and the respective FGF22- and FGFR-competent control mice (white bars) before (‘Pre’) and 2 (‘2 wks’) and 3 (‘3 wks’) weeks after a spinal cord injury (n = 7–10 animals per group). *P < 0.05, **P < 0.01 (repeated-measure ANOVA followed by Bonferroni tests).
Image of a spinal cord injured mouse walking on the catwalk that assesses locomotor recovery. Illumination of the paws from below allows to determine the paw angle body axis by relating the axis of the paw (line shown magnified in the right panel) to the axis of the body (line shown in left panel).
Quantification of the paw angle body axis of the hindpaws in FGF22-deficient (top panel, red bars) and hindlimb motor cortex FGFR1/FGFR2 double-deficient (bottom panel, blue bars) mice and respective FGF22- and FGFR-competent control mice (white bars) before (‘Pre’) and 2 (‘2 wks’) and 3 (‘3 wks’) weeks after a spinal cord injury. Between 13 and 40 steps were analyzed per group and timepoint (n = 10–15 animals per group). **P < 0.01, ***P < 0.001 (two-way ANOVA followed by Bonferroni tests).
Similar articles
-
Distinct sets of FGF receptors sculpt excitatory and inhibitory synaptogenesis.Development. 2015 May 15;142(10):1818-30. doi: 10.1242/dev.115568. Epub 2015 Apr 29. Development. 2015. PMID: 25926357 Free PMC article.
-
Synaptogenic gene therapy with FGF22 improves circuit plasticity and functional recovery following spinal cord injury.EMBO Mol Med. 2023 Feb 8;15(2):e16111. doi: 10.15252/emmm.202216111. Epub 2023 Jan 5. EMBO Mol Med. 2023. PMID: 36601738 Free PMC article.
-
AAV-KLF7 Promotes Descending Propriospinal Neuron Axonal Plasticity after Spinal Cord Injury.Neural Plast. 2017;2017:1621629. doi: 10.1155/2017/1621629. Epub 2017 Aug 13. Neural Plast. 2017. PMID: 28884027 Free PMC article.
-
Determinants of Axon Growth, Plasticity, and Regeneration in the Context of Spinal Cord Injury.Am J Pathol. 2018 Jan;188(1):53-62. doi: 10.1016/j.ajpath.2017.09.005. Epub 2017 Oct 10. Am J Pathol. 2018. PMID: 29030051 Free PMC article. Review.
-
The Function of FGFR1 Signalling in the Spinal Cord: Therapeutic Approaches Using FGFR1 Ligands after Spinal Cord Injury.Neural Plast. 2017;2017:2740768. doi: 10.1155/2017/2740768. Epub 2017 Jan 18. Neural Plast. 2017. PMID: 28197342 Free PMC article. Review.
Cited by
-
Role of FGF System in Neuroendocrine Neoplasms: Potential Therapeutic Applications.Front Endocrinol (Lausanne). 2021 Apr 14;12:665631. doi: 10.3389/fendo.2021.665631. eCollection 2021. Front Endocrinol (Lausanne). 2021. PMID: 33935975 Free PMC article. Review.
-
Age and Sex-Related Changes to Gene Expression in the Mouse Spinal Cord.J Mol Neurosci. 2019 Nov;69(3):419-432. doi: 10.1007/s12031-019-01371-3. Epub 2019 Jul 2. J Mol Neurosci. 2019. PMID: 31267314
-
FGF22 deletion causes hidden hearing loss by affecting the function of inner hair cell ribbon synapses.Front Mol Neurosci. 2022 Jul 28;15:922665. doi: 10.3389/fnmol.2022.922665. eCollection 2022. Front Mol Neurosci. 2022. PMID: 35966010 Free PMC article.
-
Panoramic RNA expression of fibroblast growth factors in human glioblastoma tissues and the impact on the survival of patients.Oncol Lett. 2024 May 14;28(1):317. doi: 10.3892/ol.2024.14450. eCollection 2024 Jul. Oncol Lett. 2024. PMID: 38807663 Free PMC article.
-
Required growth facilitators propel axon regeneration across complete spinal cord injury.Nature. 2018 Sep;561(7723):396-400. doi: 10.1038/s41586-018-0467-6. Epub 2018 Aug 29. Nature. 2018. PMID: 30158698 Free PMC article.
References
-
- Bareyre FM, Kerschensteiner M, Raineteau O, Mettenleiter TC, Weinmann O, Schwab ME. The injured spinal cord spontaneously forms a new intraspinal circuit in adult rats. Nat Neurosci. 2004;7:269–277. - PubMed
-
- Bareyre FM, Kerschensteiner M, Misgeld T, Sanes JR. Transgenic labeling of the corticospinal tract for monitoring axonal responses to spinal cord injury. Nat Med. 2005;11:1355–1360. - PubMed
-
- van den Brand R, Heutschi J, Barraud Q, DiGiovanna J, Bartholdi K, Huerlimann M, Friedli L, Vollenweider I, Moraud EM, Duis S, Dominici N, Micera S, Musienko P, Courtine G. Restoring voluntary control of locomotion after paralyzing spinal cord injury. Science. 2012;336:1182–1185. - PubMed
-
- Fox MA, Sanes JR, Borza DB, Eswarakumar VP, Fassler R, Hudson BG, John SW, Ninomiya Y, Pedchenko V, Pfaff SL, Rheault MN, Sado Y, Segal Y, Werle MJ, Umemori H. Distinct target-derived signals organize formation, maturation, and maintenance of motor nerve terminals. Cell. 2007;129:179–193. - PubMed
Publication types
MeSH terms
Substances
Grants and funding
LinkOut - more resources
Full Text Sources
Other Literature Sources
Medical
Molecular Biology Databases
Miscellaneous