Use of in vivo magnetic resonance spectroscopy for studying metabolic diseases
- PMID: 25656949
- PMCID: PMC4346484
- DOI: 10.1038/emm.2014.101
Use of in vivo magnetic resonance spectroscopy for studying metabolic diseases
Abstract
Owing to the worldwide obesity epidemic and the sedentary lifestyle in industrialized countries, the number of people with metabolic diseases is explosively increasing. Magnetic resonance spectroscopy (MRS), which is fundamentally similar to magnetic resonance imaging, can detect metabolic changes in vivo noninvasively. With its noninvasive nature, (1)H, (13)C and (31)P MRS are being actively utilized in clinical and biomedical metabolic studies to detect lipids and important metabolites without ionizing radiation. (1)H MRS can quantify lipid content in liver and muscle and can detect other metabolites, such as 2-hydroxyglutarate, in vivo. Of interest, many studies have indicated that hepatic and intramyocellular lipid content is inversely correlated with insulin sensitivity in humans. Thus, lipid content can be utilized as an in vivo biomarker for detecting early insulin resistance. Employing (13)C MRS, hepatic glycogen synthesis and breakdown can be directly detected, whereas (31)P MRS provides in vivo adenosine triphosphate (ATP) synthesis rates by saturation transfer methods in addition to ATP content. These in vivo data can be very difficult to assess by other methods and offer a critical piece of metabolic information. To aid the reader in understanding these new methods, fundamentals of MRS are described in this review in addition to promising future applications of MRS and its limitations.
Figures
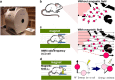
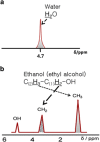
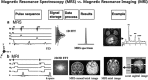
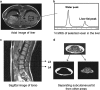
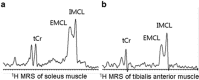
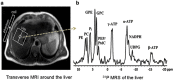
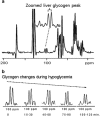
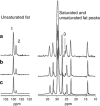
Similar articles
-
Development and applications of in vivo clinical magnetic resonance spectroscopy.Prog Biophys Mol Biol. 1996;65(1-2):45-81. doi: 10.1016/s0079-6107(96)00006-5. Prog Biophys Mol Biol. 1996. PMID: 9029941 Review.
-
Observation of intramyocellular lipids by 1H-magnetic resonance spectroscopy.Ann N Y Acad Sci. 2000 May;904:25-31. Ann N Y Acad Sci. 2000. PMID: 10865706 Review.
-
What might be the impact on neurology of the analysis of brain metabolism by in vivo magnetic resonance spectroscopy?J Neurol. 1994 May;241(6):354-71. doi: 10.1007/BF02033352. J Neurol. 1994. PMID: 7931430 Review.
-
Quantitative ATP synthesis in human liver measured by localized 31P spectroscopy using the magnetization transfer experiment.NMR Biomed. 2008 Jun;21(5):437-43. doi: 10.1002/nbm.1207. NMR Biomed. 2008. PMID: 17910026
-
Application of Magnetic Resonance Spectroscopy in metabolic research.Biochim Biophys Acta Mol Basis Dis. 2019 Apr 1;1865(4):741-748. doi: 10.1016/j.bbadis.2018.09.013. Epub 2018 Sep 24. Biochim Biophys Acta Mol Basis Dis. 2019. PMID: 30261288 Review.
Cited by
-
Label-Free Chemically and Molecularly Selective Magnetic Resonance Imaging.Chem Biomed Imaging. 2023 Apr 12;1(2):121-139. doi: 10.1021/cbmi.3c00019. eCollection 2023 May 22. Chem Biomed Imaging. 2023. PMID: 37235188 Free PMC article. Review.
-
Quantitative imaging of lipid droplets in single cells.Analyst. 2019 Jan 28;144(3):753-765. doi: 10.1039/c8an01525b. Analyst. 2019. PMID: 30357117 Free PMC article. Review.
-
Quantum approximate Bayesian computation for NMR model inference.Nat Mach Intell. 2020 Jul;2(7):396-402. doi: 10.1038/s42256-020-0198-x. Epub 2020 Jul 6. Nat Mach Intell. 2020. PMID: 33163858 Free PMC article.
-
Magnetic resonance imaging and spectroscopy for differential assessment of liver abnormalities induced by Opisthorchis felineus in an animal model.PLoS Negl Trop Dis. 2017 Jul 14;11(7):e0005778. doi: 10.1371/journal.pntd.0005778. eCollection 2017 Jul. PLoS Negl Trop Dis. 2017. PMID: 28708894 Free PMC article.
-
Tracing insights into human metabolism using chemical engineering approaches.Curr Opin Chem Eng. 2016 Nov;14:72-81. doi: 10.1016/j.coche.2016.08.019. Epub 2016 Sep 10. Curr Opin Chem Eng. 2016. PMID: 28480159 Free PMC article.
References
-
- Hwang J-H, Stein DT, Barzilai N, Cui MH, Tonelli J, Kishore P, et al. Increased intrahepatic triglyceride is associated with peripheral insulin resistance: in vivo MR imaging and spectroscopy studies. Am J Physiol Endocrinol Metab. 2007;293:E1663–E1669. - PubMed
-
- Szczepaniak LS, Babcock EE, Schick F, Dobbins RL, Garg A, Burns DK, et al. Measurement of intracellular triglyceride stores by H spectroscopy: validation in vivo. Am J Physiol Endocrinol Metab. 1999;276:E977–E989. - PubMed
-
- Yki-Järvinen H. Liver fat in the pathogenesis of insulin resistance and type 2 diabetes. Dig Dis. 2010;28:203–209. - PubMed
-
- Lauterbur PC. Image formation by induced local interaction: examples employing nuclear magnetic resonance. Nature. 1973;242:190–191. - PubMed
-
- Mansfield P, Grannell PK. NMR 'diffraction' in solids. J Phys C Solid State Phys. 1973;6:L422–L426.
Publication types
MeSH terms
Substances
LinkOut - more resources
Full Text Sources
Other Literature Sources
Medical