Chemistry and biology of the initial steps in vision: the Friedenwald lecture
- PMID: 25338686
- PMCID: PMC4207101
- DOI: 10.1167/iovs.14-15502
Chemistry and biology of the initial steps in vision: the Friedenwald lecture
Abstract
Visual transduction is the process in the eye whereby absorption of light in the retina is translated into electrical signals that ultimately reach the brain. The first challenge presented by visual transduction is to understand its molecular basis. We know that maintenance of vision is a continuous process requiring the activation and subsequent restoration of a vitamin A-derived chromophore through a series of chemical reactions catalyzed by enzymes in the retina and retinal pigment epithelium (RPE). Diverse biochemical approaches that identified key proteins and reactions were essential to achieve a mechanistic understanding of these visual processes. The three-dimensional arrangements of these enzymes' polypeptide chains provide invaluable insights into their mechanisms of action. A wealth of information has already been obtained by solving high-resolution crystal structures of both rhodopsin and the retinoid isomerase from pigment RPE (RPE65). Rhodopsin, which is activated by photoisomerization of its 11-cis-retinylidene chromophore, is a prototypical member of a large family of membrane-bound proteins called G protein-coupled receptors (GPCRs). RPE65 is a retinoid isomerase critical for regeneration of the chromophore. Electron microscopy (EM) and atomic force microscopy have provided insights into how certain proteins are assembled to form much larger structures such as rod photoreceptor cell outer segment membranes. A second challenge of visual transduction is to use this knowledge to devise therapeutic approaches that can prevent or reverse conditions leading to blindness. Imaging modalities like optical coherence tomography (OCT) and scanning laser ophthalmoscopy (SLO) applied to appropriate animal models as well as human retinal imaging have been employed to characterize blinding diseases, monitor their progression, and evaluate the success of therapeutic agents. Lately two-photon (2-PO) imaging, together with biochemical assays, are revealing functional aspects of vision at a new molecular level. These multidisciplinary approaches combined with suitable animal models and inbred mutant species can be especially helpful in translating provocative cell and tissue culture findings into therapeutic options for further development in animals and eventually in humans. A host of different approaches and techniques is required for substantial progress in understanding fundamental properties of the visual system.
Keywords: G protein–coupled receptor(s); membrane proteins; photoreceptors; phototransduction; protein structure; receptor phosphorylation; rhodopsin; signal transduction; vision.
Copyright 2014 The Association for Research in Vision and Ophthalmology, Inc.
Figures
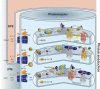
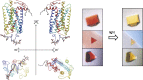
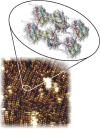
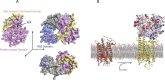
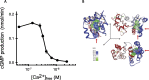
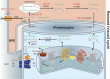
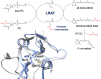
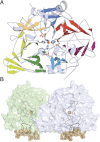
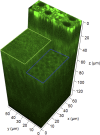
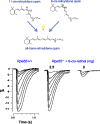
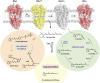
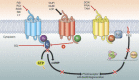
Similar articles
-
Confronting complexity: the interlink of phototransduction and retinoid metabolism in the vertebrate retina.Prog Retin Eye Res. 2001 Jul;20(4):469-529. doi: 10.1016/s1350-9462(01)00002-7. Prog Retin Eye Res. 2001. PMID: 11390257 Review.
-
Vitamin A and Vision.Subcell Biochem. 2016;81:231-259. doi: 10.1007/978-94-024-0945-1_9. Subcell Biochem. 2016. PMID: 27830507 Review.
-
Key enzymes of the retinoid (visual) cycle in vertebrate retina.Biochim Biophys Acta. 2012 Jan;1821(1):137-51. doi: 10.1016/j.bbalip.2011.03.005. Epub 2011 Apr 5. Biochim Biophys Acta. 2012. PMID: 21447403 Free PMC article. Review.
-
Photophysiological functions of visual pigments.Adv Biophys. 1984;17:5-67. doi: 10.1016/0065-227x(84)90024-8. Adv Biophys. 1984. PMID: 6242325 Review.
-
Analysis of the retinoid isomerase activities in the retinal pigment epithelium and retina.Methods Mol Biol. 2010;652:329-39. doi: 10.1007/978-1-60327-325-1_19. Methods Mol Biol. 2010. PMID: 20552438 Free PMC article.
Cited by
-
Single-residue posttranslational modification sites at the N-terminus, C-terminus or in-between: To be or not to be exposed for enzyme access.Proteomics. 2015 Jul;15(14):2525-46. doi: 10.1002/pmic.201400633. Proteomics. 2015. PMID: 26038108 Free PMC article.
-
Amplification of olfactory transduction currents implements sparse stimulus encoding.bioRxiv [Preprint]. 2024 Oct 12:2024.10.11.617893. doi: 10.1101/2024.10.11.617893. bioRxiv. 2024. PMID: 39416025 Free PMC article. Preprint.
-
Development of chiral fluorinated alkyl derivatives of emixustat as drug candidates for the treatment of retinal degenerative diseases.Bioorg Med Chem Lett. 2020 Sep 15;30(18):127421. doi: 10.1016/j.bmcl.2020.127421. Epub 2020 Jul 18. Bioorg Med Chem Lett. 2020. PMID: 32717613 Free PMC article.
-
Differential expression of the five redox complexes in the retinal mitochondria or rod outer segment disks is consistent with their different functionality.FASEB Bioadv. 2020 Mar 31;2(5):315-324. doi: 10.1096/fba.2019-00093. eCollection 2020 May. FASEB Bioadv. 2020. PMID: 32395704 Free PMC article.
-
Specialized Cilia in Mammalian Sensory Systems.Cells. 2015 Sep 11;4(3):500-19. doi: 10.3390/cells4030500. Cells. 2015. PMID: 26378583 Free PMC article. Review.
References
-
- Arshavsky VY, Lamb TD, Pugh EN Jr. G proteins and phototransduction. Annu Rev Physiol. 2002; 64: 153–187 - PubMed
Publication types
MeSH terms
Substances
Grants and funding
LinkOut - more resources
Full Text Sources
Other Literature Sources