State-of-the-art of 3D cultures (organs-on-a-chip) in safety testing and pathophysiology
- PMID: 25027500
- PMCID: PMC4783151
- DOI: 10.14573/altex.1406111
State-of-the-art of 3D cultures (organs-on-a-chip) in safety testing and pathophysiology
Abstract
Integrated approaches using different in vitro methods in combination with bioinformatics can (i) increase the success rate and speed of drug development; (ii) improve the accuracy of toxicological risk assessment; and (iii) increase our understanding of disease. Three-dimensional (3D) cell culture models are important building blocks of this strategy which has emerged during the last years. The majority of these models are organotypic, i.e., they aim to reproduce major functions of an organ or organ system. This implies in many cases that more than one cell type forms the 3D structure, and often matrix elements play an important role. This review summarizes the state of the art concerning commonalities of the different models. For instance, the theory of mass transport/metabolite exchange in 3D systems and the special analytical requirements for test endpoints in organotypic cultures are discussed in detail. In the next part, 3D model systems for selected organs--liver, lung, skin, brain--are presented and characterized in dedicated chapters. Also, 3D approaches to the modeling of tumors are presented and discussed. All chapters give a historical background, illustrate the large variety of approaches, and highlight up- and downsides as well as specific requirements. Moreover, they refer to the application in disease modeling, drug discovery and safety assessment. Finally, consensus recommendations indicate a roadmap for the successful implementation of 3D models in routine screening. It is expected that the use of such models will accelerate progress by reducing error rates and wrong predictions from compound testing.
Figures
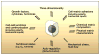
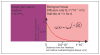
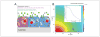
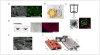
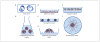
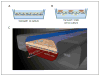
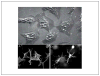
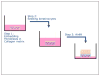
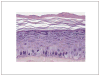
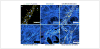
Similar articles
-
Tissue models: a living system on a chip.Nature. 2011 Mar 31;471(7340):661-5. doi: 10.1038/471661a. Nature. 2011. PMID: 21455183 No abstract available.
-
In Vitro Modeling of Idiopathic Pulmonary Fibrosis: Lung-on-a-Chip Systems and Other 3D Cultures.Int J Mol Sci. 2024 Nov 1;25(21):11751. doi: 10.3390/ijms252111751. Int J Mol Sci. 2024. PMID: 39519302 Free PMC article. Review.
-
The future of the patient-specific Body-on-a-chip.Lab Chip. 2013 Sep 21;13(18):3471-80. doi: 10.1039/c3lc50237f. Lab Chip. 2013. PMID: 23685915 Review.
-
Systems biology for organotypic cell cultures.ALTEX. 2017;34(2):301-310. doi: 10.14573/altex.1608221. Epub 2016 Nov 14. ALTEX. 2017. PMID: 27846345
-
Implementing organ-on-chip in a next-generation risk assessment of chemicals: a review.Arch Toxicol. 2022 Mar;96(3):711-741. doi: 10.1007/s00204-022-03234-0. Epub 2022 Feb 1. Arch Toxicol. 2022. PMID: 35103818 Free PMC article. Review.
Cited by
-
Organophosphorus flame retardants are developmental neurotoxicants in a rat primary brainsphere in vitro model.Arch Toxicol. 2021 Jan;95(1):207-228. doi: 10.1007/s00204-020-02903-2. Epub 2020 Oct 19. Arch Toxicol. 2021. PMID: 33078273 Free PMC article.
-
Air-Liquid Interface In Vitro Models for Respiratory Toxicology Research: Consensus Workshop and Recommendations.Appl In Vitro Toxicol. 2018 Jun 1;4(2):91-106. doi: 10.1089/aivt.2017.0034. Appl In Vitro Toxicol. 2018. PMID: 32953944 Free PMC article. Review.
-
Optical tissue clearing associated with 3D imaging: application in preclinical and clinical studies.Histochem Cell Biol. 2022 May;157(5):497-511. doi: 10.1007/s00418-022-02081-5. Epub 2022 Mar 2. Histochem Cell Biol. 2022. PMID: 35235045 Free PMC article. Review.
-
Human Induced Pluripotent Stem Cell-Derived 3D-Neurospheres are Suitable for Neurotoxicity Screening.Cells. 2020 May 1;9(5):1122. doi: 10.3390/cells9051122. Cells. 2020. PMID: 32369990 Free PMC article.
-
Harnessing the power of novel animal-free test methods for the development of COVID-19 drugs and vaccines.Arch Toxicol. 2020 Jun;94(6):2263-2272. doi: 10.1007/s00204-020-02787-2. Epub 2020 May 23. Arch Toxicol. 2020. PMID: 32447523 Free PMC article.
References
-
- Aardema MJ, Barnett BC, Khambatta Z, et al. International prevalidation studies of the EpiDerm 3D human reconstructed skin micronucleus (RSMN) assay: Transferability and reproducibility. Mutat Res. 2010;701:123–131. http://dx.doi.org/10.1016/j.mrgentox.2010.05.017. - DOI - PubMed
-
- Aardema MJ, Barnett BB, Mun GC, et al. Evaluation of chemicals requiring metabolic activation in the EpiDerm 3D human reconstructed skin micronucleus (RSMN) assay. Mutat Res. 2013;750:40–49. http://dx.doi.org/10.1016/j.mrgentox.2012.08.009. - DOI - PubMed
-
- Abbott A. Cell culture: Biology’s new dimension. Nature. 2003;424:870–872. http://dx.doi.org/10.1038/424870a. - DOI - PubMed
-
- Ackermann K, Borgia SL, Korting HC, et al. The Phenion full-thickness skin model for percutaneous absorption testing. Skin Pharmacol Physiol. 2010;23:105–112. http://dx.doi.org/10.1159/000265681. - DOI - PubMed
-
- Ahlenius H, Kokaia Z. Isolation and generation of neurosphere cultures from embryonic and adult mouse brain. Methods Mol Biol. 2010;633:241–252. http://dx.doi.org/10.1007/978-1-59745-019-5_18. - DOI - PubMed
Publication types
MeSH terms
Grants and funding
LinkOut - more resources
Full Text Sources
Other Literature Sources