The physiology of mechanoelectrical transduction channels in hearing
- PMID: 24987009
- PMCID: PMC4101631
- DOI: 10.1152/physrev.00038.2013
The physiology of mechanoelectrical transduction channels in hearing
Abstract
Much is known about the mechanotransducer (MT) channels mediating transduction in hair cells of the vertrbrate inner ear. With the use of isolated preparations, it is experimentally feasible to deliver precise mechanical stimuli to individual cells and record the ensuing transducer currents. This approach has shown that small (1-100 nm) deflections of the hair-cell stereociliary bundle are transmitted via interciliary tip links to open MT channels at the tops of the stereocilia. These channels are cation-permeable with a high selectivity for Ca(2+); two channels are thought to be localized at the lower end of the tip link, each with a large single-channel conductance that increases from the low- to high-frequency end of the cochlea. Ca(2+) influx through open channels regulates their resting open probability, which may contribute to setting the hair cell resting potential in vivo. Ca(2+) also controls transducer fast adaptation and force generation by the hair bundle, the two coupled processes increasing in speed from cochlear apex to base. The molecular intricacy of the stereocilary bundle and the transduction apparatus is reflected by the large number of single-gene mutations that are linked to sensorineural deafness, especially those in Usher syndrome. Studies of such mutants have led to the discovery of many of the molecules of the transduction complex, including the tip link and its attachments to the stereociliary core. However, the MT channel protein is still not firmly identified, nor is it known whether the channel is activated by force delivered through accessory proteins or by deformation of the lipid bilayer.
Copyright © 2014 the American Physiological Society.
Figures
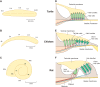
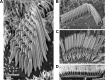
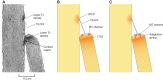
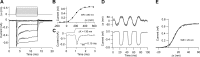
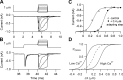
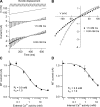
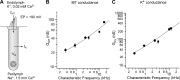
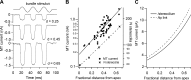
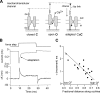
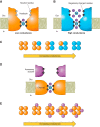
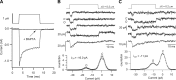
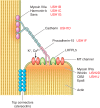
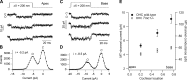
Similar articles
-
The contribution of TMC1 to adaptation of mechanoelectrical transduction channels in cochlear outer hair cells.J Physiol. 2019 Dec;597(24):5949-5961. doi: 10.1113/JP278799. Epub 2019 Nov 12. J Physiol. 2019. PMID: 31633194 Free PMC article.
-
Subunit determination of the conductance of hair-cell mechanotransducer channels.Proc Natl Acad Sci U S A. 2015 Feb 3;112(5):1589-94. doi: 10.1073/pnas.1420906112. Epub 2014 Dec 30. Proc Natl Acad Sci U S A. 2015. PMID: 25550511 Free PMC article.
-
Conductance and block of hair-cell mechanotransducer channels in transmembrane channel-like protein mutants.J Gen Physiol. 2014 Jul;144(1):55-69. doi: 10.1085/jgp.201411173. J Gen Physiol. 2014. PMID: 24981230 Free PMC article.
-
PIEZO2 as the anomalous mechanotransducer channel in auditory hair cells.J Physiol. 2017 Dec 1;595(23):7039-7048. doi: 10.1113/JP274996. Epub 2017 Oct 26. J Physiol. 2017. PMID: 28983916 Free PMC article. Review.
-
Localisation of the mechanotransducer channels in mammalian cochlear hair cells provides clues to their gating.J Physiol. 2010 Mar 1;588(Pt 5):765-72. doi: 10.1113/jphysiol.2009.179614. Epub 2009 Dec 21. J Physiol. 2010. PMID: 20026619 Free PMC article. Review.
Cited by
-
The structure of the Caenorhabditis elegans TMC-2 complex suggests roles of lipid-mediated subunit contacts in mechanosensory transduction.Proc Natl Acad Sci U S A. 2024 Feb 20;121(8):e2314096121. doi: 10.1073/pnas.2314096121. Epub 2024 Feb 14. Proc Natl Acad Sci U S A. 2024. PMID: 38354260 Free PMC article.
-
Fast adaptation of cooperative channels engenders Hopf bifurcations in auditory hair cells.Biophys J. 2022 Mar 15;121(6):897-909. doi: 10.1016/j.bpj.2022.02.016. Epub 2022 Feb 15. Biophys J. 2022. PMID: 35176272 Free PMC article.
-
Dextran Labeling and Uptake in Live and Functional Murine Cochlear Hair Cells.J Vis Exp. 2020 Feb 8;(156):10.3791/60769. doi: 10.3791/60769. J Vis Exp. 2020. PMID: 32090986 Free PMC article.
-
TRPV6, TRPM6 and TRPM7 Do Not Contribute to Hair-Cell Mechanotransduction.Front Cell Neurosci. 2018 Feb 20;12:41. doi: 10.3389/fncel.2018.00041. eCollection 2018. Front Cell Neurosci. 2018. PMID: 29515374 Free PMC article.
-
Wbp2 is required for normal glutamatergic synapses in the cochlea and is crucial for hearing.EMBO Mol Med. 2016 Mar 1;8(3):191-207. doi: 10.15252/emmm.201505523. EMBO Mol Med. 2016. PMID: 26881968 Free PMC article.
References
-
- Ahmed ZM, Goodyear R, Riazuddin S, Lagziel A, Legan PK, Behra M, Burgess SM, Lilley KS, Wilcox ER, Riazuddin S, Griffith AJ, Frolenkov GI, Belyantseva IA, Richardson GP, Friedman TB. The tip-link antigen, a protein associated with the transduction complex of sensory hair cells, is protocadherin-15. J Neurosci 26: 7022–7034, 2006 - PMC - PubMed
Publication types
MeSH terms
Grants and funding
LinkOut - more resources
Full Text Sources
Other Literature Sources
Miscellaneous