Mouse SLX4 is a tumor suppressor that stimulates the activity of the nuclease XPF-ERCC1 in DNA crosslink repair
- PMID: 24726326
- PMCID: PMC4017094
- DOI: 10.1016/j.molcel.2014.03.014
Mouse SLX4 is a tumor suppressor that stimulates the activity of the nuclease XPF-ERCC1 in DNA crosslink repair
Abstract
SLX4 binds to three nucleases (XPF-ERCC1, MUS81-EME1, and SLX1), and its deficiency leads to genomic instability, sensitivity to DNA crosslinking agents, and Fanconi anemia. However, it is not understood how SLX4 and its associated nucleases act in DNA crosslink repair. Here, we uncover consequences of mouse Slx4 deficiency and reveal its function in DNA crosslink repair. Slx4-deficient mice develop epithelial cancers and have a contracted hematopoietic stem cell pool. The N-terminal domain of SLX4 (mini-SLX4) that only binds to XPF-ERCC1 is sufficient to confer resistance to DNA crosslinking agents. Recombinant mini-SLX4 enhances XPF-ERCC1 nuclease activity up to 100-fold, directing specificity toward DNA forks. Mini-SLX4-XPF-ERCC1 also vigorously stimulates dual incisions around a DNA crosslink embedded in a synthetic replication fork, an essential step in the repair of this lesion. These observations define vertebrate SLX4 as a tumor suppressor, which activates XPF-ERCC1 nuclease specificity in DNA crosslink repair.
Copyright © 2014 The Authors. Published by Elsevier Inc. All rights reserved.
Figures
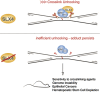
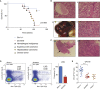
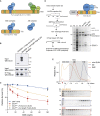
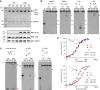
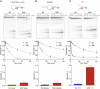
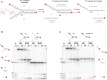

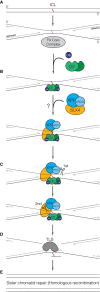
Similar articles
-
The SMX DNA Repair Tri-nuclease.Mol Cell. 2017 Mar 2;65(5):848-860.e11. doi: 10.1016/j.molcel.2017.01.031. Mol Cell. 2017. PMID: 28257701 Free PMC article.
-
The role of SLX4 and its associated nucleases in DNA interstrand crosslink repair.Nucleic Acids Res. 2019 Mar 18;47(5):2377-2388. doi: 10.1093/nar/gky1276. Nucleic Acids Res. 2019. PMID: 30576517 Free PMC article.
-
Coordination of structure-specific nucleases by human SLX4/BTBD12 is required for DNA repair.Mol Cell. 2009 Jul 10;35(1):116-27. doi: 10.1016/j.molcel.2009.06.020. Mol Cell. 2009. PMID: 19595721
-
The ERCC1 and ERCC4 (XPF) genes and gene products.Gene. 2015 Sep 15;569(2):153-61. doi: 10.1016/j.gene.2015.06.026. Epub 2015 Jun 12. Gene. 2015. PMID: 26074087 Free PMC article. Review.
-
Orchestrating the nucleases involved in DNA interstrand cross-link (ICL) repair.Cell Cycle. 2011 Dec 1;10(23):3999-4008. doi: 10.4161/cc.10.23.18385. Epub 2011 Dec 1. Cell Cycle. 2011. PMID: 22101340 Free PMC article. Review.
Cited by
-
Inhibitors of the Fanconi anaemia pathway as potential antitumour agents for ovarian cancer.Explor Target Antitumor Ther. 2020;1(1):26-52. doi: 10.37349/etat.2020.00003. Epub 2020 Feb 29. Explor Target Antitumor Ther. 2020. PMID: 36046263 Free PMC article. Review.
-
The functional status of DNA repair pathways determines the sensitization effect to cisplatin in non-small cell lung cancer cells.Cell Oncol (Dordr). 2016 Dec;39(6):511-522. doi: 10.1007/s13402-016-0291-7. Epub 2016 Jul 29. Cell Oncol (Dordr). 2016. PMID: 27473273
-
Persistent DNA damage-induced NLRP12 improves hematopoietic stem cell function.JCI Insight. 2020 May 21;5(10):e133365. doi: 10.1172/jci.insight.133365. JCI Insight. 2020. PMID: 32434992 Free PMC article.
-
A role for the base excision repair enzyme NEIL3 in replication-dependent repair of interstrand DNA cross-links derived from psoralen and abasic sites.DNA Repair (Amst). 2017 Apr;52:1-11. doi: 10.1016/j.dnarep.2017.02.011. Epub 2017 Feb 20. DNA Repair (Amst). 2017. PMID: 28262582 Free PMC article. Review.
-
Crosstalk between DNA Damage Repair and Metabolic Regulation in Hematopoietic Stem Cells.Cells. 2024 Apr 24;13(9):733. doi: 10.3390/cells13090733. Cells. 2024. PMID: 38727270 Free PMC article. Review.
References
Publication types
MeSH terms
Substances
Grants and funding
LinkOut - more resources
Full Text Sources
Other Literature Sources
Molecular Biology Databases