Thiol-based redox switches
- PMID: 24657586
- PMCID: PMC4059413
- DOI: 10.1016/j.bbapap.2014.03.007
Thiol-based redox switches
Abstract
Regulation of protein function through thiol-based redox switches plays an important role in the response and adaptation to local and global changes in the cellular levels of reactive oxygen species (ROS). Redox regulation is used by first responder proteins, such as ROS-specific transcriptional regulators, chaperones or metabolic enzymes to protect cells against mounting levels of oxidants, repair the damage and restore redox homeostasis. Redox regulation of phosphatases and kinases is used to control the activity of select eukaryotic signaling pathways, making reactive oxygen species important second messengers that regulate growth, development and differentiation. In this review we will compare different types of reversible protein thiol modifications, elaborate on their structural and functional consequences and discuss their role in oxidative stress response and ROS adaptation. This article is part of a Special Issue entitled: Thiol-Based Redox Processes.
Keywords: Disulfide bond; Oxidative stress; Redox regulation; Sulfenic acid.
Copyright © 2014 Elsevier B.V. All rights reserved.
Figures
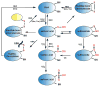
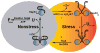
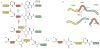
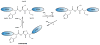
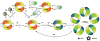
Similar articles
-
Oxidative stress, thiols, and redox profiles.Methods Mol Biol. 2012;889:325-46. doi: 10.1007/978-1-61779-867-2_21. Methods Mol Biol. 2012. PMID: 22669675
-
Mitochondrial respiratory chain complexes as sources and targets of thiol-based redox-regulation.Biochim Biophys Acta. 2014 Aug;1844(8):1344-54. doi: 10.1016/j.bbapap.2014.02.006. Epub 2014 Feb 19. Biochim Biophys Acta. 2014. PMID: 24561273 Review.
-
Plant Chloroplast Stress Response: Insights from Thiol Redox Proteomics.Antioxid Redox Signal. 2020 Jul 1;33(1):35-57. doi: 10.1089/ars.2019.7823. Epub 2020 Mar 12. Antioxid Redox Signal. 2020. PMID: 31989831 Review.
-
Thiol-based redox switches and gene regulation.Antioxid Redox Signal. 2011 Mar 15;14(6):1049-63. doi: 10.1089/ars.2010.3400. Epub 2010 Oct 28. Antioxid Redox Signal. 2011. PMID: 20626317 Free PMC article. Review.
-
The redox regulation of thiol dependent signaling pathways in cancer.Curr Pharm Des. 2006;12(34):4427-43. doi: 10.2174/138161206779010549. Curr Pharm Des. 2006. PMID: 17168752 Review.
Cited by
-
Oxidative Stress from Environmental Exposures.Curr Opin Toxicol. 2018 Feb 20;7:60-66. Curr Opin Toxicol. 2018. PMID: 30079382 Free PMC article.
-
How the assembly and protection of the bacterial cell envelope depend on cysteine residues.J Biol Chem. 2020 Aug 21;295(34):11984-11994. doi: 10.1074/jbc.REV120.011201. Epub 2020 Jun 2. J Biol Chem. 2020. PMID: 32487747 Free PMC article. Review.
-
Simulation of gap junction formation reveals critical role of Cys disulfide redox state in connexin hemichannel docking.Cell Commun Signal. 2024 Mar 18;22(1):185. doi: 10.1186/s12964-023-01439-z. Cell Commun Signal. 2024. PMID: 38500186 Free PMC article.
-
About the dangers, costs and benefits of living an aerobic lifestyle.Biochem Soc Trans. 2014 Aug;42(4):917-21. doi: 10.1042/BST20140108. Biochem Soc Trans. 2014. PMID: 25109979 Free PMC article. Review.
-
Protein Sulfenylation: A Novel Readout of Environmental Oxidant Stress.Chem Res Toxicol. 2015 Dec 21;28(12):2411-8. doi: 10.1021/acs.chemrestox.5b00424. Epub 2015 Dec 7. Chem Res Toxicol. 2015. PMID: 26605980 Free PMC article.
References
-
- Stadtman ER. Protein oxidation in aging and age-related diseases. Ann N Y Acad Sci. 2001;928:22–38. - PubMed
-
- Cooke MS, et al. Oxidative DNA damage: mechanisms, mutation, and disease. FASEB J. 2003;17(10):1195–214. - PubMed
-
- Bader N, Grune T. Protein oxidation and proteolysis. Biol Chem. 2006;387(10–11):1351–5. - PubMed
Publication types
MeSH terms
Substances
Grants and funding
LinkOut - more resources
Full Text Sources
Other Literature Sources
Molecular Biology Databases