Dodecyl maltoside protects membrane proteins in vacuo
- PMID: 23931313
- PMCID: PMC3736684
- DOI: 10.1016/j.bpj.2013.06.025
Dodecyl maltoside protects membrane proteins in vacuo
Abstract
Molecular dynamics simulations have been used to characterize the effects of transfer from aqueous solution to a vacuum to inform our understanding of mass spectrometry of membrane-protein-detergent complexes. We compared two membrane protein architectures (an α-helical bundle versus a β-barrel) and two different detergent types (phosphocholines versus an alkyl sugar) with respect to protein stability and detergent packing. The β-barrel membrane protein remained stable as a protein-detergent complex in vacuum. Zwitterionic detergents formed conformationally destabilizing interactions with an α-helical membrane protein after detergent micelle inversion driven by dehydration in vacuum. In contrast, a nonionic alkyl sugar detergent resisted micelle inversion, maintaining the solution-phase conformation of the protein. This helps to explain the relative stability of membrane proteins in the presence of alkyl sugar detergents such as dodecyl maltoside.
Copyright © 2013 The Authors. Published by Elsevier Inc. All rights reserved.
Figures
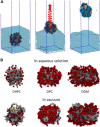
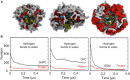
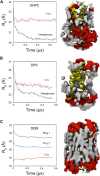
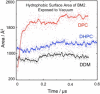
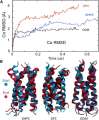
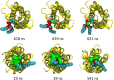
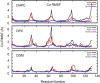
Similar articles
-
Interactions of lipids and detergents with a viral ion channel protein: molecular dynamics simulation studies.J Phys Chem B. 2015 Jan 22;119(3):764-72. doi: 10.1021/jp505127y. Epub 2014 Oct 21. J Phys Chem B. 2015. PMID: 25286030 Free PMC article.
-
New Malonate-Derived Tetraglucoside Detergents for Membrane Protein Stability.ACS Chem Biol. 2020 Jun 19;15(6):1697-1707. doi: 10.1021/acschembio.0c00316. Epub 2020 Jun 5. ACS Chem Biol. 2020. PMID: 32501004 Free PMC article.
-
Tryptophan octyl ester in detergent micelles of dodecylmaltoside: fluorescence properties and quenching by brominated detergent analogs.Biophys J. 1999 Dec;77(6):3071-84. doi: 10.1016/S0006-3495(99)77138-9. Biophys J. 1999. PMID: 10585929 Free PMC article.
-
Determination of the molecular mass and dimensions of membrane proteins by size exclusion chromatography.Methods. 2008 Oct;46(2):62-72. doi: 10.1016/j.ymeth.2008.10.020. Epub 2008 Oct 24. Methods. 2008. PMID: 18952172 Review.
-
Detergent-mediated protein aggregation.Chem Phys Lipids. 2013 Apr;169:72-84. doi: 10.1016/j.chemphyslip.2013.02.005. Epub 2013 Mar 4. Chem Phys Lipids. 2013. PMID: 23466535 Free PMC article. Review.
Cited by
-
Histatin-1 is an endogenous ligand of the sigma-2 receptor.FEBS J. 2021 Dec;288(23):6815-6827. doi: 10.1111/febs.16108. Epub 2021 Jul 23. FEBS J. 2021. PMID: 34233061 Free PMC article.
-
Solution NMR investigations of integral membrane proteins: Challenges and innovations.Curr Opin Struct Biol. 2023 Oct;82:102654. doi: 10.1016/j.sbi.2023.102654. Epub 2023 Aug 3. Curr Opin Struct Biol. 2023. PMID: 37542910 Free PMC article. Review.
-
Interactions of lipids and detergents with a viral ion channel protein: molecular dynamics simulation studies.J Phys Chem B. 2015 Jan 22;119(3):764-72. doi: 10.1021/jp505127y. Epub 2014 Oct 21. J Phys Chem B. 2015. PMID: 25286030 Free PMC article.
-
FPOP-LC-MS/MS Suggests Differences in Interaction Sites of Amphipols and Detergents with Outer Membrane Proteins.J Am Soc Mass Spectrom. 2017 Jan;28(1):50-55. doi: 10.1007/s13361-016-1421-1. Epub 2016 Jun 24. J Am Soc Mass Spectrom. 2017. PMID: 27343183 Free PMC article.
-
Amphipols outperform dodecylmaltoside micelles in stabilizing membrane protein structure in the gas phase.Anal Chem. 2015 Jan 20;87(2):1118-26. doi: 10.1021/ac5037022. Epub 2014 Dec 30. Anal Chem. 2015. PMID: 25495802 Free PMC article.
References
-
- White S.H. Biophysical dissection of membrane proteins. Nature. 2009;459:344–346. - PubMed
Publication types
MeSH terms
Substances
Grants and funding
- WT_/Wellcome Trust/United Kingdom
- BBS/B/16011/BB_/Biotechnology and Biological Sciences Research Council/United Kingdom
- BB/H000267/1/BB_/Biotechnology and Biological Sciences Research Council/United Kingdom
- BEP17032/BB_/Biotechnology and Biological Sciences Research Council/United Kingdom
- BB/I019855/1/BB_/Biotechnology and Biological Sciences Research Council/United Kingdom
LinkOut - more resources
Full Text Sources
Other Literature Sources