The core microprocessor component DiGeorge syndrome critical region 8 (DGCR8) is a nonspecific RNA-binding protein
- PMID: 23893406
- PMCID: PMC3772224
- DOI: 10.1074/jbc.M112.446880
The core microprocessor component DiGeorge syndrome critical region 8 (DGCR8) is a nonspecific RNA-binding protein
Abstract
MicroRNA (miRNA) biogenesis follows a conserved succession of processing steps, beginning with the recognition and liberation of an miRNA-containing precursor miRNA hairpin from a large primary miRNA transcript (pri-miRNA) by the Microprocessor, which consists of the nuclear RNase III Drosha and the double-stranded RNA-binding domain protein DGCR8 (DiGeorge syndrome critical region protein 8). Current models suggest that specific recognition is driven by DGCR8 detection of single-stranded elements of the pri-miRNA stem-loop followed by Drosha recruitment and pri-miRNA cleavage. Because countless RNA transcripts feature single-stranded-dsRNA junctions and DGCR8 can bind hundreds of mRNAs, we explored correlations between RNA binding properties of DGCR8 and specific pri-miRNA substrate processing. We found that DGCR8 bound single-stranded, double-stranded, and random hairpin transcripts with similar affinity. Further investigation of DGCR8/pri-mir-16 interactions by NMR detected intermediate exchange regimes over a wide range of stoichiometric ratios. Diffusion analysis of DGCR8/pri-mir-16 interactions by pulsed field gradient NMR lent further support to dynamic complex formation involving free components in exchange with complexes of varying stoichiometry, although in vitro processing assays showed exclusive cleavage of pri-mir-16 variants bearing single-stranded flanking regions. Our results indicate that DGCR8 binds RNA nonspecifically. Therefore, a sequential model of DGCR8 recognition followed by Drosha recruitment is unlikely. Known RNA substrate requirements are broad and include 70-nucleotide hairpins with unpaired flanking regions. Thus, specific RNA processing is likely facilitated by preformed DGCR8-Drosha heterodimers that can discriminate between authentic substrates and other hairpins.
Keywords: DGCR8; Double-stranded RNA-binding Domain (dsRBD); Drosha; MicroRNA; Microprocessor; NMR; Protein-Nucleic Acid Interaction; RNA Structure; RNA-binding Protein.
Figures
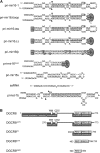
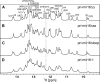
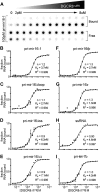
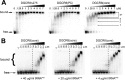
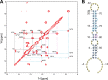
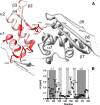
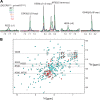
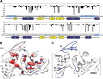
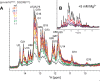
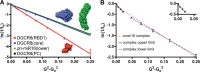
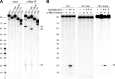
Similar articles
-
A heterotrimer model of the complete Microprocessor complex revealed by single-molecule subunit counting.RNA. 2016 Feb;22(2):175-83. doi: 10.1261/rna.054684.115. Epub 2015 Dec 18. RNA. 2016. PMID: 26683315 Free PMC article.
-
Deformability in the cleavage site of primary microRNA is not sensed by the double-stranded RNA binding domains in the microprocessor component DGCR8.Proteins. 2015 Jun;83(6):1165-79. doi: 10.1002/prot.24810. Epub 2015 Apr 28. Proteins. 2015. PMID: 25851436 Free PMC article.
-
Structural Basis for pri-miRNA Recognition by Drosha.Mol Cell. 2020 May 7;78(3):423-433.e5. doi: 10.1016/j.molcel.2020.02.024. Epub 2020 Mar 27. Mol Cell. 2020. PMID: 32220645
-
MicroRNA biogenesis: isolation and characterization of the microprocessor complex.Methods Mol Biol. 2006;342:33-47. doi: 10.1385/1-59745-123-1:33. Methods Mol Biol. 2006. PMID: 16957365 Review.
-
Cellular functions of the microprocessor.Biochem Soc Trans. 2013 Aug;41(4):838-43. doi: 10.1042/BST20130011. Biochem Soc Trans. 2013. PMID: 23863141 Review.
Cited by
-
Binding by TRBP-dsRBD2 Does Not Induce Bending of Double-Stranded RNA.Biophys J. 2016 Jun 21;110(12):2610-2617. doi: 10.1016/j.bpj.2016.05.012. Biophys J. 2016. PMID: 27332119 Free PMC article.
-
Stabilizing heterochromatin by DGCR8 alleviates senescence and osteoarthritis.Nat Commun. 2019 Jul 26;10(1):3329. doi: 10.1038/s41467-019-10831-8. Nat Commun. 2019. PMID: 31350386 Free PMC article.
-
The Regulatory Role of MicroRNAs in Breast Cancer.Int J Mol Sci. 2019 Oct 6;20(19):4940. doi: 10.3390/ijms20194940. Int J Mol Sci. 2019. PMID: 31590453 Free PMC article. Review.
-
DGCR8 Acts as an Adaptor for the Exosome Complex to Degrade Double-Stranded Structured RNAs.Mol Cell. 2015 Dec 17;60(6):873-85. doi: 10.1016/j.molcel.2015.11.011. Epub 2015 Dec 10. Mol Cell. 2015. PMID: 26687677 Free PMC article.
-
Macrocyclization of a Ligand Targeting a Toxic RNA Dramatically Improves Potency.Chembiochem. 2020 Nov 16;21(22):3229-3233. doi: 10.1002/cbic.202000445. Epub 2020 Aug 26. Chembiochem. 2020. PMID: 32649032 Free PMC article.
References
Publication types
MeSH terms
Substances
LinkOut - more resources
Full Text Sources
Other Literature Sources