The pharmacology of regenerative medicine
- PMID: 23818131
- PMCID: PMC3698935
- DOI: 10.1124/pr.112.007393
The pharmacology of regenerative medicine
Abstract
Regenerative medicine is a rapidly evolving multidisciplinary, translational research enterprise whose explicit purpose is to advance technologies for the repair and replacement of damaged cells, tissues, and organs. Scientific progress in the field has been steady and expectations for its robust clinical application continue to rise. The major thesis of this review is that the pharmacological sciences will contribute critically to the accelerated translational progress and clinical utility of regenerative medicine technologies. In 2007, we coined the phrase "regenerative pharmacology" to describe the enormous possibilities that could occur at the interface between pharmacology, regenerative medicine, and tissue engineering. The operational definition of regenerative pharmacology is "the application of pharmacological sciences to accelerate, optimize, and characterize (either in vitro or in vivo) the development, maturation, and function of bioengineered and regenerating tissues." As such, regenerative pharmacology seeks to cure disease through restoration of tissue/organ function. This strategy is distinct from standard pharmacotherapy, which is often limited to the amelioration of symptoms. Our goal here is to get pharmacologists more involved in this field of research by exposing them to the tools, opportunities, challenges, and interdisciplinary expertise that will be required to ensure awareness and galvanize involvement. To this end, we illustrate ways in which the pharmacological sciences can drive future innovations in regenerative medicine and tissue engineering and thus help to revolutionize the discovery of curative therapeutics. Hopefully, the broad foundational knowledge provided herein will spark sustained conversations among experts in diverse fields of scientific research to the benefit of all.
Figures
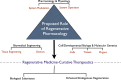
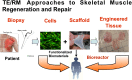
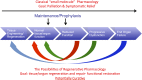
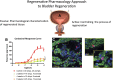
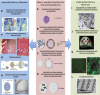
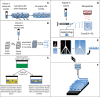
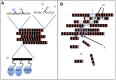
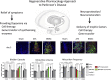
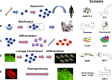
Similar articles
-
Regenerative pharmacology: recent developments and future perspectives.Regen Med. 2016 Dec;11(8):859-870. doi: 10.2217/rme-2016-0108. Epub 2016 Dec 1. Regen Med. 2016. PMID: 27905259 Review.
-
Regenerative pharmacology: the future is now.Mol Interv. 2007 Apr;7(2):79-86. doi: 10.1124/mi.7.2.8. Mol Interv. 2007. PMID: 17468388 Review.
-
Regenerative medicine in urology.Semin Pediatr Surg. 2014 Jun;23(3):106-11. doi: 10.1053/j.sempedsurg.2014.05.002. Epub 2014 May 22. Semin Pediatr Surg. 2014. PMID: 24994523 Review.
-
Advent and maturation of regenerative medicine.Curr Stem Cell Res Ther. 2012 Nov;7(6):430-45. doi: 10.2174/157488812804484657. Curr Stem Cell Res Ther. 2012. PMID: 23176292 Review.
-
Bench to bedside: Current advances in regenerative medicine.Curr Opin Cell Biol. 2018 Dec;55:59-66. doi: 10.1016/j.ceb.2018.05.006. Epub 2018 Jul 11. Curr Opin Cell Biol. 2018. PMID: 30007127 Review.
Cited by
-
Modulating the Biomechanical Properties of Engineered Connective Tissues by Chitosan-Coated Multiwall Carbon Nanotubes.Int J Nanomedicine. 2021 Feb 15;16:989-1000. doi: 10.2147/IJN.S289107. eCollection 2021. Int J Nanomedicine. 2021. PMID: 33633447 Free PMC article.
-
Current Status of Alginate in Drug Delivery.Adv Pharmacol Pharm Sci. 2020 Aug 6;2020:8886095. doi: 10.1155/2020/8886095. eCollection 2020. Adv Pharmacol Pharm Sci. 2020. PMID: 32832902 Free PMC article. Review.
-
The Progression of Regenerative Medicine and its Impact on Therapy Translation.Clin Transl Sci. 2020 May;13(3):440-450. doi: 10.1111/cts.12736. Epub 2020 Feb 6. Clin Transl Sci. 2020. PMID: 31981408 Free PMC article. Review.
-
Cellular Mechanisms of Liver Regeneration and Cell-Based Therapies of Liver Diseases.Biomed Res Int. 2017;2017:8910821. doi: 10.1155/2017/8910821. Epub 2017 Jan 22. Biomed Res Int. 2017. PMID: 28210629 Free PMC article. Review.
-
Collagen-Based Biomaterials in Periodontal Regeneration: Current Applications and Future Perspectives of Plant-Based Collagen.Biomimetics (Basel). 2022 Mar 24;7(2):34. doi: 10.3390/biomimetics7020034. Biomimetics (Basel). 2022. PMID: 35466251 Free PMC article. Review.
References
-
- Andersson KE, Chapple CR, Cardozo L, Cruz F, Hashim H, Michel MC, Tannenbaum C, Wein AJ. (2009) Pharmacological treatment of overactive bladder: report from the International Consultation on Incontinence. Curr Opin Urol 19:380–394 - PubMed
-
- Andersson KE, Christ GJ. (2007) Regenerative pharmacology: the future is now. Mol Interv 7:79–86 - PubMed
-
- Aron L, Klein R. (2011) Repairing the parkinsonian brain with neurotrophic factors. Trends Neurosci 34:88–100 - PubMed
Publication types
MeSH terms
Substances
Grants and funding
LinkOut - more resources
Full Text Sources
Other Literature Sources
Research Materials