Physiological temperature during brain slicing enhances the quality of acute slice preparations
- PMID: 23630465
- PMCID: PMC3632751
- DOI: 10.3389/fncel.2013.00048
Physiological temperature during brain slicing enhances the quality of acute slice preparations
Abstract
We demonstrate that brain dissection and slicing using solutions warmed to near-physiological temperature (~ +34°C), greatly enhance slice quality without affecting intrinsic electrophysiological properties of the neurons. Improved slice quality is seen not only when using young (<1 month), but also mature (>2.5 month) mice. This allows easy in vitro patch-clamp experimentation using adult deep cerebellar nuclear slices, which until now have been considered very difficult. As proof of the concept, we compare intrinsic properties of cerebellar nuclear neurons in juvenile (<1 month) and adult (up to 7 months) mice, and confirm that no significant developmental changes occur after the fourth postnatal week. The enhanced quality of brain slices from old animals facilitates experimentation on age-related disorders as well as optogenetic studies requiring long transfection periods.
Keywords: acute slice preparation; cerebellar nuclei; cerebellum; mature animals.
Figures
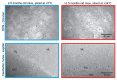
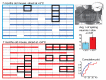
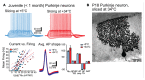
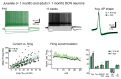
Similar articles
-
Acute Cerebellar Slice Preparation Using a Tissue Chopper.Bio Protoc. 2019 Mar 5;9(5):e3187. doi: 10.21769/BioProtoc.3187. eCollection 2019 Mar 5. Bio Protoc. 2019. PMID: 33654989 Free PMC article.
-
Slice it hot: acute adult brain slicing in physiological temperature.J Vis Exp. 2014 Oct 30;(92):e52068. doi: 10.3791/52068. J Vis Exp. 2014. PMID: 25406663 Free PMC article.
-
Advantages of Acute Brain Slices Prepared at Physiological Temperature in the Characterization of Synaptic Functions.Front Cell Neurosci. 2020 Mar 19;14:63. doi: 10.3389/fncel.2020.00063. eCollection 2020. Front Cell Neurosci. 2020. PMID: 32265664 Free PMC article.
-
The mouse cerebellar cortex in organotypic slice cultures: an in vitro model to analyze the consequences of mutations and pathologies on neuronal survival, development, and function.Crit Rev Neurobiol. 2006;18(1-2):179-86. doi: 10.1615/critrevneurobiol.v18.i1-2.180. Crit Rev Neurobiol. 2006. PMID: 17725520 Review.
-
Time window control: a model for cerebellar function based on synchronization, reverberation, and time slicing.Prog Brain Res. 2000;124:275-97. doi: 10.1016/S0079-6123(00)24023-5. Prog Brain Res. 2000. PMID: 10943132 Review.
Cited by
-
Mystixin-7 Peptide Protects Ionotropic Glutamatergic Mechanisms against Glutamate-Induced Excitotoxicity In Vitro.Int J Pept. 2016;2016:5151843. doi: 10.1155/2016/5151843. Epub 2016 Jul 18. Int J Pept. 2016. PMID: 27504123 Free PMC article.
-
Functional mitochondrial analysis in acute brain sections from adult rats reveals mitochondrial dysfunction in a rat model of migraine.Am J Physiol Cell Physiol. 2014 Dec 1;307(11):C1017-30. doi: 10.1152/ajpcell.00332.2013. Epub 2014 Sep 24. Am J Physiol Cell Physiol. 2014. PMID: 25252946 Free PMC article.
-
A high-density multi-electrode platform examining the effects of radiation on in vitro cortical networks.Sci Rep. 2024 Aug 29;14(1):20143. doi: 10.1038/s41598-024-71038-6. Sci Rep. 2024. PMID: 39210021 Free PMC article.
-
Single Exposure to Cocaine Impairs Reinforcement Learning by Potentiating the Activity of Neurons in the Direct Striatal Pathway in Mice.Neurosci Bull. 2021 Aug;37(8):1119-1134. doi: 10.1007/s12264-021-00687-8. Epub 2021 Apr 27. Neurosci Bull. 2021. PMID: 33905097 Free PMC article.
-
Barrel cortex VIP/ChAT interneurons suppress sensory responses in vivo.PLoS Biol. 2020 Feb 6;18(2):e3000613. doi: 10.1371/journal.pbio.3000613. eCollection 2020 Feb. PLoS Biol. 2020. PMID: 32027647 Free PMC article.
References
-
- Aizenman C. D., Linden D. J. (1999). Regulation of the rebound depolarization and spontaneous firing patterns of deep nuclear neurons in slices of rat cerebellum. J. Neurophysiol. 82 1697–1709 - PubMed
-
- Aminov Y. (2010). P2S2 – “Ping-Pong” Spike Sorting, An Interactive Method for Spike Sorting Using Markov Chain Monte Carlo. Master Thesis, Ben Gurion University of the Negev; Israel
-
- Andersen P. (1981). Brain slices — a neurobiological tool of increasing usefulness. Trends Neurosci. 4 53–56
LinkOut - more resources
Full Text Sources
Other Literature Sources
Research Materials