A phenotypic change but not proliferation underlies glial responses in Alzheimer disease
- PMID: 23602650
- PMCID: PMC3668030
- DOI: 10.1016/j.ajpath.2013.02.031
A phenotypic change but not proliferation underlies glial responses in Alzheimer disease
Abstract
Classical immunohistochemical studies in the Alzheimer disease (AD) brain reveal prominent glial reactions, but whether this pathological feature is due primarily to cell proliferation or to a phenotypic change of existing resting cells remains controversial. We performed double-fluorescence immunohistochemical studies of astrocytes and microglia, followed by unbiased stereology-based quantitation in temporal cortex of 40 AD patients and 32 age-matched nondemented subjects. Glial fibrillary acidic protein (GFAP) and major histocompatibility complex II (MHC2) were used as markers of astrocytic and microglial activation, respectively. Aldehyde dehydrogenase 1 L1 and glutamine synthetase were used as constitutive astrocytic markers, and ionized calcium-binding adaptor molecule 1 (IBA1) as a constitutive microglial marker. As expected, AD patients had higher numbers of GFAP(+) astrocytes and MHC2(+) microglia than the nondemented subjects. However, both groups had similar numbers of total astrocytes and microglia and, in the AD group, these total numbers remained essentially constant over the clinical course of the disease. The GFAP immunoreactivity of astrocytes, but not the MHC2 immunoreactivity of microglia, increased in parallel with the duration of the clinical illness in the AD group. Cortical atrophy contributed to the perception of increased glia density. We conclude that a phenotypic change of existing glial cells, rather than a marked proliferation of glial precursors, accounts for the majority of the glial responses observed in the AD brain.
Copyright © 2013 American Society for Investigative Pathology. Published by Elsevier Inc. All rights reserved.
Figures
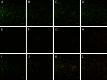
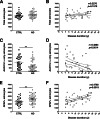
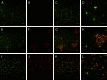
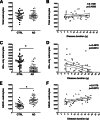
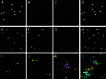
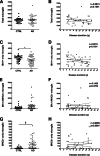



Similar articles
-
GFAP and vimentin deficiency alters gene expression in astrocytes and microglia in wild-type mice and changes the transcriptional response of reactive glia in mouse model for Alzheimer's disease.Glia. 2015 Jun;63(6):1036-56. doi: 10.1002/glia.22800. Epub 2015 Mar 2. Glia. 2015. PMID: 25731615
-
Distinct changes in all major components of the neurovascular unit across different neuropathological stages of Alzheimer's disease.Brain Pathol. 2020 Nov;30(6):1056-1070. doi: 10.1111/bpa.12895. Epub 2020 Sep 16. Brain Pathol. 2020. PMID: 32866303 Free PMC article.
-
Inflammatory pathology markers (activated microglia and reactive astrocytes) in early and late onset Alzheimer disease: a post mortem study.Neuropathol Appl Neurobiol. 2018 Apr;44(3):298-313. doi: 10.1111/nan.12445. Epub 2017 Nov 27. Neuropathol Appl Neurobiol. 2018. PMID: 29044639
-
Microglia-Astrocyte Communication in Alzheimer's Disease.J Alzheimers Dis. 2023;95(3):785-803. doi: 10.3233/JAD-230199. J Alzheimers Dis. 2023. PMID: 37638434 Free PMC article. Review.
-
Microglia and Astrocytes in Alzheimer's Disease in the Context of the Aberrant Copper Homeostasis Hypothesis.Biomolecules. 2021 Oct 28;11(11):1598. doi: 10.3390/biom11111598. Biomolecules. 2021. PMID: 34827595 Free PMC article. Review.
Cited by
-
TLR4-mediated chronic neuroinflammation has no effect on tangle pathology in a tauopathy mouse model.Front Aging Neurosci. 2024 Oct 21;16:1468602. doi: 10.3389/fnagi.2024.1468602. eCollection 2024. Front Aging Neurosci. 2024. PMID: 39503044 Free PMC article.
-
Deciphering the Astrocyte Reaction in Alzheimer's Disease.Front Aging Neurosci. 2018 Apr 25;10:114. doi: 10.3389/fnagi.2018.00114. eCollection 2018. Front Aging Neurosci. 2018. PMID: 29922147 Free PMC article. Review.
-
Immunohistological Examination of AKT Isoforms in the Brain: Cell-Type Specificity That May Underlie AKT's Role in Complex Brain Disorders and Neurological Disease.Cereb Cortex Commun. 2021 May 28;2(2):tgab036. doi: 10.1093/texcom/tgab036. eCollection 2021. Cereb Cortex Commun. 2021. PMID: 34296180 Free PMC article.
-
The microglial translocator protein (TSPO) in Alzheimer's disease reflects a phagocytic phenotype.Acta Neuropathol. 2024 Nov 14;148(1):62. doi: 10.1007/s00401-024-02822-x. Acta Neuropathol. 2024. PMID: 39540994 Free PMC article.
-
Gliovascular alterations in sporadic and familial Alzheimer's disease: APOE3 Christchurch homozygote glioprotection.Brain Pathol. 2023 Mar;33(2):e13119. doi: 10.1111/bpa.13119. Epub 2022 Sep 21. Brain Pathol. 2023. PMID: 36130084 Free PMC article.
References
-
- Beach T.G., Walker R., McGeer E.G. Patterns of gliosis in Alzheimer's disease and aging cerebrum. Glia. 1989;2:420–436. - PubMed
-
- Itagaki S., McGeer P.L., Akiyama H., Zhu S., Selkoe D. Relationship of microglia and astrocytes to amyloid deposits of Alzheimer disease. J Neuroimmunol. 1989;24:173–182. - PubMed
-
- Cahoy J.D., Emery B., Kaushal A., Foo L.C., Zamanian J.L., Christopherson K.S., Xing Y., Lubischer J.L., Krieg P.A., Krupenko S.A., Thompson W.J., Barres B.A. A transcriptome database for astrocytes, neurons, and oligodendrocytes: a new resource for understanding brain development and function. J Neurosci. 2008;28:264–278. - PMC - PubMed
-
- Ulvestad E., Williams K., Mørk S., Antel J., Nyland H. Phenotypic differences between human monocytes/macrophages and microglial cells studied in situ and in vitro. J Neuropathol Exp Neurol. 1994;53:492–501. - PubMed
Publication types
MeSH terms
Substances
Grants and funding
LinkOut - more resources
Full Text Sources
Other Literature Sources
Medical
Research Materials
Miscellaneous