Ion channel associated diseases: overview of molecular mechanisms
- PMID: 23151230
- PMCID: PMC3586387
- DOI: 10.1021/cr300360k
Ion channel associated diseases: overview of molecular mechanisms
Figures
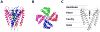
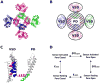
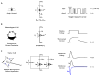
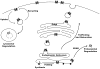
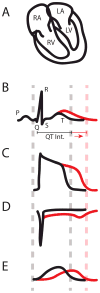
Similar articles
-
SnapShot: Channel Gating Mechanisms.Cell. 2017 Jul 27;170(3):594-594.e1. doi: 10.1016/j.cell.2017.07.019. Cell. 2017. PMID: 28753432
-
From molecule to malady.Nature. 2006 Mar 23;440(7083):440-7. doi: 10.1038/nature04707. Nature. 2006. PMID: 16554803 Review.
-
Structure and function of voltage-gated ion channels.Annu Rev Biochem. 1995;64:493-531. doi: 10.1146/annurev.bi.64.070195.002425. Annu Rev Biochem. 1995. PMID: 7574491 Review.
-
Ion channel-directed therapies in autonomic neurons: a view from the bench.Clin Auton Res. 2002 Apr;12(2):59-65. doi: 10.1007/s102860200021. Clin Auton Res. 2002. PMID: 12102451 Review.
-
Engineering light-gated ion channels.Biochemistry. 2006 Dec 26;45(51):15129-41. doi: 10.1021/bi0618058. Epub 2006 Dec 2. Biochemistry. 2006. PMID: 17176035 Review.
Cited by
-
Electronic Polarization Leads to a Drier Dewetted State for Hydrophobic Gating in the Big Potassium Channel.J Phys Chem Lett. 2024 Jul 25;15(29):7436-7441. doi: 10.1021/acs.jpclett.4c01359. Epub 2024 Jul 15. J Phys Chem Lett. 2024. PMID: 39008088
-
Inner pore hydration free energy controls the activation of big potassium channels.Biophys J. 2023 Apr 4;122(7):1158-1167. doi: 10.1016/j.bpj.2023.02.005. Epub 2023 Feb 10. Biophys J. 2023. PMID: 36774534 Free PMC article.
-
Recent Advances in Computer-Aided Structure-Based Drug Design on Ion Channels.Int J Mol Sci. 2023 May 25;24(11):9226. doi: 10.3390/ijms24119226. Int J Mol Sci. 2023. PMID: 37298178 Free PMC article. Review.
-
A computational model of organism development and carcinogenesis resulting from cells' bioelectric properties and communication.Sci Rep. 2022 Jun 2;12(1):9206. doi: 10.1038/s41598-022-13281-3. Sci Rep. 2022. PMID: 35654933 Free PMC article.
-
Waixenicin A, a marine-derived TRPM7 inhibitor: a promising CNS drug lead.Acta Pharmacol Sin. 2020 Dec;41(12):1519-1524. doi: 10.1038/s41401-020-00512-4. Epub 2020 Sep 29. Acta Pharmacol Sin. 2020. PMID: 32994545 Free PMC article. Review.
References
-
- Armstrong CM, Hille B. Neuron. 1998;20:371. - PubMed
-
- Yang J, Ellinor PT, Sather WA, Zhang JF, Tsien RW. Nature. 1993;366:158. - PubMed
-
- Doyle DA, Morais Cabral J, Pfuetzner RA, Kuo A, Gulbis JM, Cohen SL, Chait BT, MacKinnon R. Science. 1998;280:69. - PubMed
-
- Zhou Y, Morais-Cabral JH, Kaufman A, MacKinnon R. Nature. 2001;414:43. - PubMed
-
- MacKinnon R. Angewandte Chemie. 2004;43:4265. - PubMed
Publication types
MeSH terms
Substances
Grants and funding
LinkOut - more resources
Full Text Sources
Other Literature Sources