Structure and function of biotin-dependent carboxylases
- PMID: 22869039
- PMCID: PMC3508090
- DOI: 10.1007/s00018-012-1096-0
Structure and function of biotin-dependent carboxylases
Abstract
Biotin-dependent carboxylases include acetyl-CoA carboxylase (ACC), propionyl-CoA carboxylase (PCC), 3-methylcrotonyl-CoA carboxylase (MCC), geranyl-CoA carboxylase, pyruvate carboxylase (PC), and urea carboxylase (UC). They contain biotin carboxylase (BC), carboxyltransferase (CT), and biotin-carboxyl carrier protein components. These enzymes are widely distributed in nature and have important functions in fatty acid metabolism, amino acid metabolism, carbohydrate metabolism, polyketide biosynthesis, urea utilization, and other cellular processes. ACCs are also attractive targets for drug discovery against type 2 diabetes, obesity, cancer, microbial infections, and other diseases, and the plastid ACC of grasses is the target of action of three classes of commercial herbicides. Deficiencies in the activities of PCC, MCC, or PC are linked to serious diseases in humans. Our understanding of these enzymes has been greatly enhanced over the past few years by the crystal structures of the holoenzymes of PCC, MCC, PC, and UC. The structures reveal unanticipated features in the architectures of the holoenzymes, including the presence of previously unrecognized domains, and provide a molecular basis for understanding their catalytic mechanism as well as the large collection of disease-causing mutations in PCC, MCC, and PC. This review will summarize the recent advances in our knowledge on the structure and function of these important metabolic enzymes.
Figures
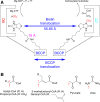
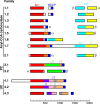
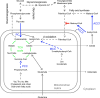
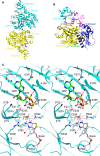
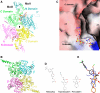
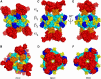
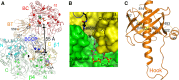
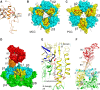
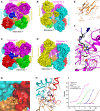
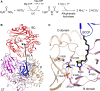
Similar articles
-
Crystal structure of the alpha(6)beta(6) holoenzyme of propionyl-coenzyme A carboxylase.Nature. 2010 Aug 19;466(7309):1001-5. doi: 10.1038/nature09302. Nature. 2010. PMID: 20725044 Free PMC article.
-
Striking Diversity in Holoenzyme Architecture and Extensive Conformational Variability in Biotin-Dependent Carboxylases.Adv Protein Chem Struct Biol. 2017;109:161-194. doi: 10.1016/bs.apcsb.2017.04.006. Epub 2017 May 23. Adv Protein Chem Struct Biol. 2017. PMID: 28683917 Review.
-
Chloroflexus aurantiacus acetyl-CoA carboxylase evolves fused biotin carboxylase and biotin carboxyl carrier protein to complete carboxylation activity.mBio. 2024 May 8;15(5):e0341423. doi: 10.1128/mbio.03414-23. Epub 2024 Apr 4. mBio. 2024. PMID: 38572988 Free PMC article.
-
Purification and characterization of mitochondrial biotin-dependent carboxylases from native tissues.Methods Enzymol. 2024;708:1-30. doi: 10.1016/bs.mie.2024.10.010. Epub 2024 Oct 28. Methods Enzymol. 2024. PMID: 39572135
-
The biotin enzyme family: conserved structural motifs and domain rearrangements.Curr Protein Pept Sci. 2003 Jun;4(3):217-29. doi: 10.2174/1389203033487199. Curr Protein Pept Sci. 2003. PMID: 12769720 Review.
Cited by
-
Fatty Acid Synthase: Structure, Function, and Regulation.Subcell Biochem. 2022;99:1-33. doi: 10.1007/978-3-031-00793-4_1. Subcell Biochem. 2022. PMID: 36151372 Review.
-
Synthesis and Evaluation of 1,3-Disubstituted Imidazolidine-2,4,5-triones as Inhibitors of Pyruvate Carboxylase.ACS Med Chem Lett. 2024 Jun 26;15(7):1088-1093. doi: 10.1021/acsmedchemlett.4c00183. eCollection 2024 Jul 11. ACS Med Chem Lett. 2024. PMID: 39015262
-
Lithium downregulates phosphorylated acetyl‑CoA carboxylase 2 and attenuates mitochondrial fatty acid utilization and oxidative stress in cardiomyocytes.Exp Ther Med. 2024 Feb 5;27(4):126. doi: 10.3892/etm.2024.12413. eCollection 2024 Apr. Exp Ther Med. 2024. PMID: 38414784 Free PMC article.
-
Biochemical and structural characterization of the BioZ enzyme engaged in bacterial biotin synthesis pathway.Nat Commun. 2021 Apr 6;12(1):2056. doi: 10.1038/s41467-021-22360-4. Nat Commun. 2021. PMID: 33824341 Free PMC article.
-
Structural insight into synergistic activation of human 3-methylcrotonyl-CoA carboxylase.Nat Struct Mol Biol. 2025 Jan;32(1):73-85. doi: 10.1038/s41594-024-01379-3. Epub 2024 Sep 2. Nat Struct Mol Biol. 2025. PMID: 39223421
References
Publication types
MeSH terms
Substances
Grants and funding
LinkOut - more resources
Full Text Sources
Other Literature Sources
Molecular Biology Databases
Miscellaneous