DNA charge transport for sensing and signaling
- PMID: 22861008
- PMCID: PMC3495616
- DOI: 10.1021/ar3001298
DNA charge transport for sensing and signaling
Abstract
The DNA duplex is an exquisite macromolecular array that stores genetic information to encode proteins and regulate pathways. Its unique structure also imparts chemical function that allows it also to mediate charge transport (CT). We have utilized diverse platforms to probe DNA CT, using spectroscopic, electrochemical, and even genetic methods. These studies have established powerful features of DNA CT chemistry. DNA CT can occur over long molecular distances as long as the bases are well stacked. The perturbations in base stacking that arise with single base mismatches, DNA lesions, and the binding of some proteins that kink the DNA all inhibit DNA CT. Significantly, single molecule studies of DNA CT show that ground state CT can occur over 34 nm if the duplex is well stacked; one single base mismatch inhibits CT. The DNA duplex is an effective sensor for the integrity of the base pair stack. Moreover, the efficiency of DNA CT is what one would expect for a stack of graphite sheets: equivalent to the stack of DNA base pairs and independent of the sugar-phosphate backbone. Since DNA CT offers a means to carry out redox chemistry from a distance, we have considered how this chemistry might be used for long range biological signaling. We have taken advantage of our chemical probes and platforms to characterize DNA CT in the context of the cell. CT can occur over long distances, perhaps funneling damage to particular sites and insulating others from oxidative stress. Significantly, transcription factors that activate the genome to respond to oxidative stress can also be activated from a distance through DNA CT. Numerous proteins maintain the integrity of the genome and an increasing number of them contain [4Fe-4S] clusters that do not appear to carry out either structural or enzymatic roles. Using electrochemical methods, we find that DNA binding shifts the redox potentials of the clusters, activating them towards oxidation at physiological potentials. We have proposed a model that describes how repair proteins may utilize DNA CT to efficiently search the genome for lesions. Importantly, many of these proteins occur in low copy numbers within the cell, and thus a processive mechanism does not provide a sufficient explanation of how they find and repair lesions before the cell divides. Using atomic force microscopy and genetic assays, we show that repair proteins proficient at DNA CT can relocalize in the vicinity of DNA lesions and can cooperate in finding lesions within the cell. Conversely, proteins defective in DNA CT cannot relocalize in the vicinity of lesions and do not assist other proteins involved in repair within the cell. Moreover such genetic defects are associated with disease in human protein analogues. As we continue to unravel this chemistry and discover more proteins with redox cofactors involved in genome maintenance, we are learning more regarding opportunities for long range signaling and sensing, and more examples of DNA CT chemistry that may provide critical functions within the cell.
Figures
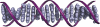
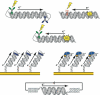
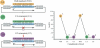
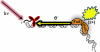
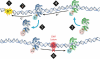
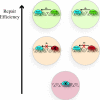
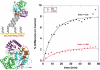
Similar articles
-
DNA-mediated charge transport in redox sensing and signaling.J Am Chem Soc. 2010 Jan 27;132(3):891-905. doi: 10.1021/ja907669c. J Am Chem Soc. 2010. PMID: 20047321 Free PMC article.
-
DNA charge transport within the cell.Biochemistry. 2015 Feb 3;54(4):962-73. doi: 10.1021/bi501520w. Epub 2015 Jan 21. Biochemistry. 2015. PMID: 25606780 Free PMC article. Review.
-
DNA charge transport as a first step in coordinating the detection of lesions by repair proteins.Proc Natl Acad Sci U S A. 2012 Feb 7;109(6):1856-61. doi: 10.1073/pnas.1120063109. Epub 2012 Jan 23. Proc Natl Acad Sci U S A. 2012. PMID: 22308447 Free PMC article.
-
Mutants of the base excision repair glycosylase, endonuclease III: DNA charge transport as a first step in lesion detection.Biochemistry. 2011 Jul 12;50(27):6133-45. doi: 10.1021/bi2003179. Epub 2011 Jun 9. Biochemistry. 2011. PMID: 21651304 Free PMC article.
-
DNA Charge Transport: from Chemical Principles to the Cell.Cell Chem Biol. 2016 Jan 21;23(1):183-197. doi: 10.1016/j.chembiol.2015.11.010. Cell Chem Biol. 2016. PMID: 26933744 Free PMC article. Review.
Cited by
-
Emerging critical roles of Fe-S clusters in DNA replication and repair.Biochim Biophys Acta. 2015 Jun;1853(6):1253-71. doi: 10.1016/j.bbamcr.2015.01.018. Epub 2015 Feb 2. Biochim Biophys Acta. 2015. PMID: 25655665 Free PMC article. Review.
-
Why Are DNA and Protein Electron Transfer So Different?Annu Rev Phys Chem. 2019 Jun 14;70:71-97. doi: 10.1146/annurev-physchem-042018-052353. Epub 2019 Feb 6. Annu Rev Phys Chem. 2019. PMID: 30726186 Free PMC article. Review.
-
Oxidation of p53 through DNA charge transport involves a network of disulfides within the DNA-binding domain.Biochemistry. 2015 Jan 27;54(3):932-41. doi: 10.1021/bi501424v. Epub 2015 Jan 13. Biochemistry. 2015. PMID: 25584637 Free PMC article.
-
How Clustered DNA Damage Can Change the Electronic Properties of ds-DNA-Differences between GAG, GAOXOG, and OXOGAOXOG.Biomolecules. 2023 Mar 11;13(3):517. doi: 10.3390/biom13030517. Biomolecules. 2023. PMID: 36979452 Free PMC article.
-
High-Precision Electrochemical Measurements of the Guanine-, Mismatch-, and Length-Dependence of Electron Transfer from Electrode-Bound DNA Are Consistent with a Contact-Mediated Mechanism.J Am Chem Soc. 2019 Jan 23;141(3):1304-1311. doi: 10.1021/jacs.8b11341. Epub 2019 Jan 11. J Am Chem Soc. 2019. PMID: 30605323 Free PMC article.
References
-
- Eley DD, Spivey DI. Semiconductivity of organic substances. Part 9. Nucleic acid in the dry state. Trans. Faraday Soc. 1962;58:411–415.
-
- Wagenknecht HA, editor. Charge Transfer in DNA: From Mechanism to Application. Wiley-VCH, Weinheim; Germany: 2005.
-
- Kelley SO, Barton JK. Electron Transfer Between Bases in Double Helical DNA. Science. 1999;283:375–381. - PubMed
Publication types
MeSH terms
Substances
Grants and funding
LinkOut - more resources
Full Text Sources
Research Materials