Cooperative cluster formation, DNA bending and base-flipping by O6-alkylguanine-DNA alkyltransferase
- PMID: 22730295
- PMCID: PMC3458534
- DOI: 10.1093/nar/gks574
Cooperative cluster formation, DNA bending and base-flipping by O6-alkylguanine-DNA alkyltransferase
Abstract
O6-Alkylguanine-DNA alkyltransferase (AGT) repairs mutagenic O6-alkylguanine and O4-alkylthymine adducts in DNA, protecting the genome and also contributing to the resistance of tumors to chemotherapeutic alkylating agents. AGT binds DNA cooperatively, and cooperative interactions are likely to be important in lesion search and repair. We examined morphologies of complexes on long, unmodified DNAs, using analytical ultracentrifugation and atomic force microscopy. AGT formed clusters of ≤11 proteins. Longer clusters, predicted by the McGhee-von Hippel model, were not seen even at high [protein]. Interestingly, torsional stress due to DNA unwinding has the potential to limit cluster size to the observed range. DNA at cluster sites showed bend angles (∼0, ∼30 and ∼60°) that are consistent with models in which each protein induces a bend of ∼30°. Distributions of complexes along the DNA are incompatible with sequence specificity but suggest modest preference for DNA ends. These properties tell us about environments in which AGT may function. Small cooperative clusters and the ability to accommodate a range of DNA bends allow function where DNA topology is constrained, such as near DNA-replication complexes. The low sequence specificity allows efficient and unbiased lesion search across the entire genome.
Figures
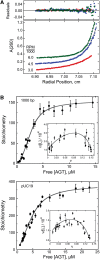
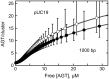


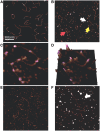
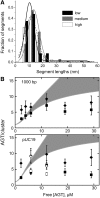

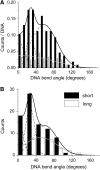
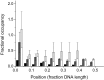
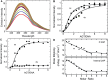
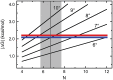
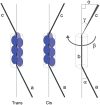
Similar articles
-
Insight into the cooperative DNA binding of the O⁶-alkylguanine DNA alkyltransferase.DNA Repair (Amst). 2014 Aug;20:14-22. doi: 10.1016/j.dnarep.2014.01.006. Epub 2014 Feb 16. DNA Repair (Amst). 2014. PMID: 24553127 Free PMC article. Review.
-
Quaternary interactions and supercoiling modulate the cooperative DNA binding of AGT.Nucleic Acids Res. 2017 Jul 7;45(12):7226-7236. doi: 10.1093/nar/gkx223. Nucleic Acids Res. 2017. PMID: 28575445 Free PMC article.
-
Interactions of human O(6)-alkylguanine-DNA alkyltransferase (AGT) with short double-stranded DNAs.Biochemistry. 2008 Dec 30;47(52):13754-63. doi: 10.1021/bi801666c. Biochemistry. 2008. PMID: 19061338 Free PMC article.
-
Interactions of human O6-alkylguanine-DNA alkyltransferase (AGT) with short single-stranded DNAs.J Biol Chem. 2007 Feb 2;282(5):3357-66. doi: 10.1074/jbc.M608876200. Epub 2006 Nov 30. J Biol Chem. 2007. PMID: 17138560 Free PMC article.
-
DNA binding, nucleotide flipping, and the helix-turn-helix motif in base repair by O6-alkylguanine-DNA alkyltransferase and its implications for cancer chemotherapy.DNA Repair (Amst). 2007 Aug 1;6(8):1100-15. doi: 10.1016/j.dnarep.2007.03.011. Epub 2007 May 7. DNA Repair (Amst). 2007. PMID: 17485252 Free PMC article. Review.
Cited by
-
Structure-function relationships governing activity and stability of a DNA alkylation damage repair thermostable protein.Nucleic Acids Res. 2015 Oct 15;43(18):8801-16. doi: 10.1093/nar/gkv774. Epub 2015 Jul 30. Nucleic Acids Res. 2015. PMID: 26227971 Free PMC article.
-
Impact of DNA sequences on DNA 'opening' by the Rad4/XPC nucleotide excision repair complex.DNA Repair (Amst). 2021 Nov;107:103194. doi: 10.1016/j.dnarep.2021.103194. Epub 2021 Jul 29. DNA Repair (Amst). 2021. PMID: 34428697 Free PMC article.
-
Studying protein-DNA interactions using atomic force microscopy.Semin Cell Dev Biol. 2018 Jan;73:220-230. doi: 10.1016/j.semcdb.2017.06.028. Epub 2017 Jun 30. Semin Cell Dev Biol. 2018. PMID: 28673677 Free PMC article. Review.
-
Strand-specific recognition of DNA damages by XPD provides insights into nucleotide excision repair substrate versatility.J Biol Chem. 2014 Feb 7;289(6):3613-24. doi: 10.1074/jbc.M113.523001. Epub 2013 Dec 14. J Biol Chem. 2014. PMID: 24338567 Free PMC article.
-
Resolving the contributions of two cooperative mechanisms to the DNA binding of AGT.Biopolymers. 2015 Sep;103(9):509-16. doi: 10.1002/bip.22684. Biopolymers. 2015. PMID: 26017689 Free PMC article.
References
-
- Pegg AE. Mammalian O6-alkylguanine-DNA alkyltransferase: regulation and importance in response to alkylating carcinogens and therapeutic agents. Cancer Res. 1990;50:6119–6129. - PubMed
Publication types
MeSH terms
Substances
Grants and funding
LinkOut - more resources
Full Text Sources
Miscellaneous