Turning the 'mustard oil bomb' into a 'cyanide bomb': aromatic glucosinolate metabolism in a specialist insect herbivore
- PMID: 22536404
- PMCID: PMC3334988
- DOI: 10.1371/journal.pone.0035545
Turning the 'mustard oil bomb' into a 'cyanide bomb': aromatic glucosinolate metabolism in a specialist insect herbivore
Abstract
Plants have evolved a variety of mechanisms for dealing with insect herbivory among which chemical defense through secondary metabolites plays a prominent role. Physiological, behavioural and sensorical adaptations to these chemicals provide herbivores with selective advantages allowing them to diversify within the newly occupied ecological niche. In turn, this may influence the evolution of plant metabolism giving rise to e.g. new chemical defenses. The association of Pierid butterflies and plants of the Brassicales has been cited as an illustrative example of this adaptive process known as 'coevolutionary armsrace'. All plants of the Brassicales are defended by the glucosinolate-myrosinase system to which larvae of cabbage white butterflies and related species are biochemically adapted through a gut nitrile-specifier protein. Here, we provide evidence by metabolite profiling and enzyme assays that metabolism of benzylglucosinolate in Pieris rapae results in release of equimolar amounts of cyanide, a potent inhibitor of cellular respiration. We further demonstrate that P. rapae larvae develop on transgenic Arabidopsis plants with ectopic production of the cyanogenic glucoside dhurrin without ill effects. Metabolite analyses and fumigation experiments indicate that cyanide is detoxified by β-cyanoalanine synthase and rhodanese in the larvae. Based on these results as well as on the facts that benzylglucosinolate was one of the predominant glucosinolates in ancient Brassicales and that ancient Brassicales lack nitrilases involved in alternative pathways, we propose that the ability of Pierid species to safely handle cyanide contributed to the primary host shift from Fabales to Brassicales that occured about 75 million years ago and was followed by Pierid species diversification.
Conflict of interest statement
Figures
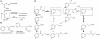
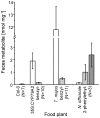
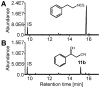
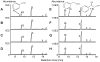
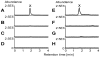
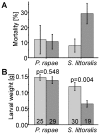
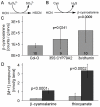
Similar articles
-
Molecular identification and characterization of rhodaneses from the insect herbivore Pieris rapae.Sci Rep. 2018 Jul 17;8(1):10819. doi: 10.1038/s41598-018-29148-5. Sci Rep. 2018. PMID: 30018390 Free PMC article.
-
Glycine conjugates in a lepidopteran insect herbivore--the metabolism of benzylglucosinolate in the cabbage white butterfly, Pieris rapae.Chembiochem. 2006 Dec;7(12):1982-9. doi: 10.1002/cbic.200600280. Chembiochem. 2006. PMID: 17086559
-
Interspecific Differences in the Larval Performance of Pieris Butterflies (Lepidoptera: Pieridae) Are Associated with Differences in the Glucosinolate Profiles of Host Plants.J Insect Sci. 2019 May 1;19(3):2. doi: 10.1093/jisesa/iez035. J Insect Sci. 2019. PMID: 31039584 Free PMC article.
-
Insect herbivore counteradaptations to the plant glucosinolate-myrosinase system.Phytochemistry. 2011 Sep;72(13):1566-75. doi: 10.1016/j.phytochem.2011.01.016. Epub 2011 Feb 10. Phytochemistry. 2011. PMID: 21316065 Review.
-
The Cellular and Subcellular Organization of the Glucosinolate-Myrosinase System against Herbivores and Pathogens.Int J Mol Sci. 2022 Jan 29;23(3):1577. doi: 10.3390/ijms23031577. Int J Mol Sci. 2022. PMID: 35163500 Free PMC article. Review.
Cited by
-
Antibiotic-producing symbionts dynamically transition between plant pathogenicity and insect-defensive mutualism.Nat Commun. 2017 Apr 28;8:15172. doi: 10.1038/ncomms15172. Nat Commun. 2017. PMID: 28452358 Free PMC article.
-
Horizontal Gene Transfer Contributes to the Evolution of Arthropod Herbivory.Genome Biol Evol. 2016 Jun 27;8(6):1785-801. doi: 10.1093/gbe/evw119. Genome Biol Evol. 2016. PMID: 27307274 Free PMC article.
-
Molecular identification and characterization of rhodaneses from the insect herbivore Pieris rapae.Sci Rep. 2018 Jul 17;8(1):10819. doi: 10.1038/s41598-018-29148-5. Sci Rep. 2018. PMID: 30018390 Free PMC article.
-
Plant Cyanogenic-Derived Metabolites and Herbivore Counter-Defences.Plants (Basel). 2024 Apr 29;13(9):1239. doi: 10.3390/plants13091239. Plants (Basel). 2024. PMID: 38732453 Free PMC article. Review.
-
Midgut serine proteases and alternative host plant utilization in Pieris brassicae L.Front Physiol. 2015 Mar 31;6:95. doi: 10.3389/fphys.2015.00095. eCollection 2015. Front Physiol. 2015. PMID: 25873901 Free PMC article.
References
-
- Wittstock U, Gershenzon J. Constitutive plant toxins and their role in plant defense. Curr Opin Plant Biol. 2002;5:300–307. - PubMed
-
- Kessler A, Halitschke R. Specificity and complexity: the impact of herbivore-induced plant responses on arthropod community structure. Curr Opin Plant Biol. 2007;10:409–414. - PubMed
-
- Hartmann T. From waste products to ecochemicals: Fifty years research of plant secondary metabolism. Phytochemistry. 2007;68:2831–2846. - PubMed
-
- Ehrlich PR, Raven PH. Butterflies and plants: a study in coevolution. Evolution. 1964;18:586–608.
-
- Becerra JX. Insects on plants: Macroevolutionary chemical trends in host use. Science. 1997;276:253–256. - PubMed
Publication types
MeSH terms
Substances
LinkOut - more resources
Full Text Sources